Research topics
The theoretical investigation of the interaction of matter with laser or other intense photon pulses by now has reached a level at which fundamental aspects such as the quantum nature of both light and matter, relativity and correlations among the involved particles have become key issues and substantial challenges alike. In this overview, our efforts are summarized towards a detailed understanding of the quantum mechanical interplay of all constituents of atomic, ionic and nuclear systems as well as quantum vacuum and quantum plasma with strong laser fields. Emphasis is placed on various applications with strong connection to experiments at the Institute (see Fig. 1): High-precision calculations involving quantum electrodynamical and nuclear effects are employed for accurate determinations, e.g., of ionic spectra and g-factors. Coherent control of atomic and nuclear ensembles is investigated in the light of advanced synchrotron and x-ray free-electron laser sources. And finally our research on extreme field laser physics is directed towards carrying out quantum electrodynamics, nuclear, high-energy and astrophysics with the strongest laser pulses presently available or under construction.
Correspondingly please find below links to our three key areas which you please click for more details on the topics, a second click will close the topic:
Precision Studies with Ions and Nuclei
High-precision experiments with ions and nuclei enable a direct access to fundamental physics. In a highly charged ion, inner-shell electrons experience the strongest electromagnetic fields accessible nowadays, providing an ideal testing ground to prove weather quantum electrodynamics, the field theory of the electromagnetic interaction, is valid in intense external fields. Precision measurements with these systems, when combined with sufficiently accurate theoretical calculations, also yield the values of fundamental constants or can test whether these vary in space and time. Such studies are also anticipated to provide access to tests of physical phenomena beyond the Standard Model of particle physics. Furthermore, nuclei become also attractive in this direction being both increasingly accessible with advanced light sources and stable against external perturbations. Due to their typical MeV energy scale, they appear attractive for applications such as compact nuclear batteries or quantum information devices.
An external static magnetic field gives rise to the Zeeman splitting of atomic energy levels, with a strength characterized by the dimensionless g-factor of the atomic state. The theory of the g-factor of an electron bound in the attractive potential of an atomic nucleus is investigated in the group around Zoltán Harman. These studies are paralleled with a quantum leap in the experimental accuracy in the investigation of the g-factor, especially in Penning trap measurements performed by the division headed by Klaus Blaum. Measuring the electron's g-factor in highly charged ions provide an exciting possibility for testing fundamental theories. Effects of strong-field quantum electrodynamics (QED) are increasingly relevant at higher and higher ionic charges (see Fig. 2). Bound-state QED effects are scrutinized to the highest precision in recent trapped-ion experiments, which have reached the 10-11 level in terms of relative precision. From a combined analysis of experimental and theoretical data for carbon ions, an improved value of the mass of the electron has been determined to world-leading accuracy. The ALPHATRAP Penning-trap setup also enables the experimental investigation of highly charged ions in virtually arbitrary charge states, which, in collaboration with theoretical calculations, largely enriches the field of applications. Penning-trap measurements have very recently also enabled a novel determination of electronic binding energies. The achieved experimental accuracy on the 1eV precision level is matched by our large-scale atomic structure calculations. Applications of this collaboration include the discovery of ultranarrow ionic transitions suitable for constructing future atomic clocks, and the determination of the Q value of the β decay of various atomic isotopes, relevant for the determination of the neutrino mass.
The g-factor experiments are currently being extended to a range of ions along the periodic table, including the heaviest stable elements. An essential motivation of such studies is that g-factor measurements with highly charged ions are anticipated to yield a new value of the fine-structure constant, i.e. the fundamental constant defining the strength of any kind of electromagnetic interactions in the Universe. We have found that a combination of the experimental g-factors of light one- and three-electron ions allows one to substract detrimental nuclear uncertainties, and thus one can extract this constant to unprecedented precision. This necessitates also a continuous improvement in the theoretical description of electron correlation effects and radiative corrections. In recent studies, we put forward the use of high-precision measurements of the g-factor of few-electron ions and its isotope shifts as a probe for physics beyond the standard model. The contribution of a hypothetical fifth fundamental force is calculated, and we found that, combining measurements from different ions at accuracy levels projected to be accessible in the near future, one may constrain the new physics coupling constant competitively. This idea was implemented in a recent experiment performed with ALPHATRAP, in which the difference between the g factors of two Ne isotopes was measured with a remarkable precision (see Fig. 3). The combination of theory and experiment provided a novel way of proving the existence of a hypothetical exotic interaction. Furthermore, our theory also enables to extract the magnetic properties of He-3 from high-precision experimental data, relevant for the calibration of new nuclear magnetic resonance probes.
Optical and x-ray spectroscopy of highly charged ions for testing fundamental theories
Highly charged ions do not only allow the precision determination of the values of physical constants, but they have also been proposed as improved optical clocks, and ideal systems for testing a potential variation in time of fundamental constants. Open-shell ions near level crossings have been predicted to be particularly sensitive to a variation of the fine-structure constant, and their optical and extreme ultraviolet spectra are being investigated by ion trap experiments by the group of José Crespo López-Urrutia. We perform large-scale calculations of the atomic structural properties of these ions to accompany the experiments, and put forward further ions and transition schemes for an efficient testing of the potential variation of the fine-structure constant and the electron-proton mass ratio. To extend the studies with highly charged ions, recently we also theoretically describe nuclear effects such as the hyperfine splitting of optical transitions in neutral atoms or singly charged ions, in collaboration with collinear laser spectroscopic measurements of the division of Klaus Blaum at the ISOLDE radioactive ion beam facility at CERN.
As these trapped-ion experiments are reaching extreme precision in the microwave and optical range, it would be desirable to also achieve high accuracy in a broader regime of the electromagnetic spectrum up to x-ray frequencies. While research with new x-ray free electron lasers has already produced remarkable results, the spectral qualities of these light sources are still far from those of optical lasers. In collaboration with the experimental group of Thomas Pfeifer at the Institute, we have developed x-ray pulse shaping schemes which are anticipated to decrease the bandwidth and improve the temporal coherence of the x-ray light. In recent studies, we have put forward a scheme to generate fully coherent x-ray lasers based on population inversion in highly charged ions, created by fast inner-shell photoionization using x-ray free-electron-laser pulses in a laser-produced plasma. Our numerical simulations show that one can obtain high-intensity, femtosecond x-ray pulses with bandwidths orders of magnitude narrower than in x-ray free-electron-laser pulses, at wavelengths down to the sub-ångström regime. Furthermore, Investigations have been started into the excitation dynamics of highly charged ions interacting with extreme ultraviolet (EUV) frequency combs, as such experiments are currently under construction in the Institute. We have put forward a scheme to infer the temporal coherence of EUV combs generated from intracavity high-order harmonic generation. Such short-wavelength lasers and frequency combs may be applicable in the study of x-ray quantum optics and metrology, investigating nonlinear interactions between x-rays and matter, or in high-precision spectroscopic studies in laboratory astrophysics.
The group around Natalia Oreshkina developed an accurate theoretical description of the structure of heavy muonic atoms and their corresponding x-ray emission spectra. The muons' probability density, due to their larger mass, greatly overlaps with the atomic nucleus, yielding access to the determination of nuclear parameters. As an example, in recent studies, the electric quadrupole moment of rhenium nuclei has been determined in collaboration with muonic x-ray spectroscopic measurements at the Paul Scherrer Institute in Switzerland. Since in these exotic atoms the energy scale of muonic and nuclear levels is comparable, a complex, unified treatment of muonic and nuclear structure is often mandatory in the theoretical modeling.
Access to nuclear structure with muonic atoms spectroscopy
Muonic atoms feature strong dependence on nuclear parameters and therefore can provide information about atomic nuclei. From the other hand, being essentially hydrogen-like highly-charged ions, they can be treated with well-known theoretical QED methods as applicable here, however with the much higher impact of nuclear physics. A combination of the knowledge about the level structure and experiments measuring the transition energies in muonic atoms enabled the determination of nuclear parameters like charge radii, electric quadrupole and magnetic dipole moments.
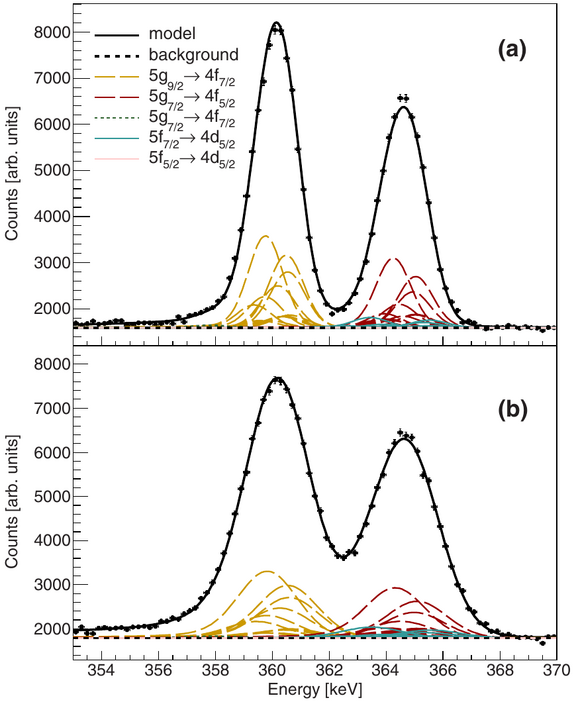
Starting with well-known effects such as finite-nuclear size, Uehling effects, and hyperfine structure, we have significantly improved the quality of heavy muonic atoms theory. Nuclear shape is included in all orders by using a deformed Fermi model, where QED and screening effects are calculated to 1 keV level as visible in current experiments. Also, dynamical splitting, a unique feature of strong mixing of nuclear and muonic levels, can be now predicted with high accuracy. Combining state-of-the-art theory predictions with measured experimental spectra of two rhenium isotopes allowed us to extract new values of the nuclear quadrupole moment (see Fig. 4), with further analysis for other nuclear parameters on the way.
To improve even further our knowledge of nuclear effects and to improve their accuracy in high-precision calculations, we use a microscopic description of nuclear dynamics. Nuclear ground-state charge distributions are obtained within the Hartree-Fock method, while complete nuclear excitation spectra are computed by means of the random-phase approximation. Then, a detailed description of nuclear dynamics is incorporated into the calculations of nuclear-polarization corrections to the energy levels of muonic atoms. Special attention is given to analyzing the nuclear model dependence, and the uncertainties of the calculations are estimated. Finally, the developed methods and computational codes were applied to the long-standing problem of the fine-structure anomalies in heavy muonic atoms. The obtained theoretical results suggest that the dominant calculation uncertainty is much smaller than the persisting discrepancies between theory and experiment, and therefore provided strong evidence against nuclear polarization being the source of the long-standing fine-structure anomaly.
X-ray Quantum Dynamics with Atoms, Ions and Nuclei
High-precision research is increasingly enriched by advanced light sources, pushing the boundaries of physical research to shorter and shorter wavelengths. However, also in its own right x-ray free electron lasers and synchrotron sources allow for novel fundamental research on correlated relativistic quantum dynamics in ions as well as on x-ray quantum optics with nuclei, and on control of nuclear forward scattering.
Highly charged ions in x-ray fields
In highly charged atomic ions, inner-shell electrons experience extremely high nuclear binding fields. The dynamics of electrons becomes relativistic. Also, the electronic probability density has a considerate overlap with the nuclear matter. These properties render these atomic systems an ideal tool for basic research in relativistic atomic structure theory, quantum electrodynamics at extreme fields, and in the study of nuclear effects. In many-electron ions, relativistic and nuclear contributions are intertwined with electron correlation. Because of this complex interplay, accurate large-scale theoretical calculations, performed by the groups around Zoltán Harman and Natalia Oreshkina, are necessary to decipher the physical phenomena from the increasingly accurate experimental spectra.
The knowledge of the structural and dynamical properties of highly charged ions is also necessary for the modeling and diagnostics of astrophysical and fusion plasmas. The spectra of inner-shell transitions are of great relevance for astrophysical line diagnostics of x-ray binaries and stars, aiming at determining physical parameters of the emitter medium such as element decomposition, density, temperature, and velocity. These transitions are predominantly in the x-ray range, therefore, their efficient radiative excitation has been rather challenging until the advent of modern x-ray sources such as free electron lasers and synchrotrons, providing high intensities in the appropriate wavelength range. Our calculations have contributed to the resolution of a conondrum that has puzzled astrophysicists for decades: The intensity of two important, strong x-ray lines of a certain highly charged iron ion in astrophysical data and in laboratory experiments did not agree with predictions, obscuring the interpretation of astronomical observations (see Fig. 5). A recent electron beam ion trap measurement at the high-brilliance PETRA III synchrotron facility finally agrees with our large-scale calculations and with other modern theoretical results.
X-ray quantum optics
Recent advances in existing and upcoming light sources provide access to laser-like light in the x-ray and gamma-ray frequency domain, with a multitude of novel applications across all the natural sciences. Nevertheless, the implementation of advanced laser-coupled quantum systems in the x-ray domain remains a challenge due to basic experimental limitations. We develop methods to overcome these limitations, and to establish x-ray quantum optics. This on the one hand will be required to unleash the full potential of the new x-ray light sources, and on the other hand provides a new platform for quantum optics as a whole.
In the group of Jörg Evers, currently the emphasis is on atomic Mössbauer nuclei, which have proven successful in ground-breaking experiments on x-ray quantum optics. A particularly promising setup is x-ray cavity quantum electrodynamics with Mössbauer nuclei embedded in thin-film cavities probed by near-resonant x-ray light. The joint nuclei-cavity system features an effective description as a tunable x-ray quantum optical few-level scheme. By choosing a suitable cavity setup, the structure of the few-level scheme, the transition frequencies and the decay rates can be controlled and changed as compared to the bare nuclear properties. Furthermore, the cavity environment can induce coherent couplings between the different states of the few-level system, thus compensating for the lack of direct x-ray control fields implemented with external sources. Over the last years, we developed a sophisticated quantum optical framework for the description of this setting. It allows identifying and separating all physical processes contributing to the recorded signal, and encompasses nonlinear and quantum effects. Based on these models, we engineer advanced quantum optical setups, and we verified a number of predictions of this model experimentally in collaboration with experimental teams around Ralf Röhlsberger (DESY, Hamburg), Thomas Pfeifer at the Institute, and G. G. Paulus (University and Helmholtz Institute Jena) at the Dynamics Beamline P01 of the synchrotron source PETRA III (DESY) and the Nuclear Resonance Beamline (ID18) of the European Synchrotron Radiation Facility (ESRF, Grenoble).
However, x-ray cavities typically have spectrally overlapping cavity modes. It has been debated in the literature whether the standard quantum optical methods apply in this case. Indeed, we found deviations between the standard quantum optical models and experimental results, and these deviations not only question the theoretical understanding, but also hinder the design of future x-ray cavity setups. Motivated by this, we recently developed ab-initio methods to derive few-mode models for quantum potential scattering problems. They rigorously connect quantum optical few-mode approaches to ab-initio scattering theory, and extends their applicability range to extreme conditions. Applying these ab initio methods to x-ray cavities resolves the discrepancies and allows for a highly efficient numerical treatment of the system. This progress then allowed us to tackle a long-standing problem: Until recently, there was no systematic way of designing x-ray cavities such that a desired few-level scheme is realized, and the range of accessible few-level schemes was unknown. We overcame this limitation by developing an inverse design approach for artificial x-ray few-level systems (see Fig. 6). It allows one to determine the required x-ray cavity structure given the desired few-level scheme. Next to the actual design, the approach also led to a number of qualitative insights into x-ray photonic environments for nuclei that will likely impact the design of future x-ray cavities and thereby improve their performance.
Correlated quantum dynamics and laser control
Mössbauer nuclei feature resonances with exceptionally narrow spectral line widths, which form the basis for a broad range of applications across the natural sciences. For x-ray quantum optics, the narrow line is favorable, because it translates into long lifetimes of the nuclear coherences. However, the narrow line width also accounts for a key challenge in nuclear quantum optics, namely, the lack of strong driving fields. The reason is that synchrotron and free-electron-laser x-ray sources deliver temporally short pulses with a broad spectrum, such that only a tiny fraction of the photons is resonant with the nuclei. To address this challenge, a group around Jörg Evers explores the possibility to simulate the effect of strong control fields using mechanical motion of a nuclear target (see Fig. 7). A short x-ray pulse impinging on a nuclear target may either pass without interaction, or interact with the nuclei. Because of the long lifetime, the interacting part of the x-ray pulse is delayed. By applying a suitable motion to the target immediately after the non-interacting part passed the target, an arbitrary time-dependent phase can be imprinted on the interacting part. While the overall phase of the x-rays varies randomly from pulse to pulse, the relative phase between the interacting and the non-interacting part can be controlled. As a first application, we demonstrated that the pulse shaping can be applied in such a way that the number of resonant x-ray photons in a given pulse can be significantly increased. Without mechanical motion, absorption reduces the number of resonant photons, because the relative phase is such that the two parts destructively interfere. Using a sudden displacement by half the x-ray wavelength, this destructive interference is turned into constructive interference, such that instead the number of resonant photons is increased. Energy is conserved, since this spectral gain on resonance is achieved by redistributing off-resonant photons onto the resonance. In the experiment with teams around Ralf Röhlsberger (DESY, Hamburg) and Thomas Pfeifer (MPIK) at the Nuclear Resonance Beamline (ID18) at ESRF, the nuclear sample was moved using a piezo film, and the sudden displacement was achieved within about 20 nanoseconds (1ns=10-9s) with a spatial precision better than one Angstrom (10-10 meter). As a result, we found an increase by more than a factor of 3, while our theoretical calculations predict a maximum possible enhancement by about one order of magnitude for the employed 57Fe nuclei. More generally, the dynamical control of the temporal phase enables one to tailor the frequency spectrum of the x-ray pulse. Sudden phase jumps lead to Fano resonances, which indicate the presence of different interfering quantum evolution pathways. This project therefore also continues a long-standing collaboration with the experimental group of Thomas Pfeifer at the Institute, in which we have established Fano resonances and in particular the interplay of its energy- and time domain interpretation as a powerful tool to investigate and also control quantum dynamics.
The shaping of the x-ray pulse spectrum using mechanical motion relies on a separation of an initial x-ray pulse into an interacting and a non-interacting part, and the motion enables one to dynamically control the relative phase between these two parts. The control possibility invites an interpretation as a tunable source of phase-coherent x-ray double-pulse sequences. This raises the question, if such double-pulses can be used to coherently control the dynamics of nuclei using suitably shaped x-ray fields. Recently, we demonstrated that this coherent x-ray-optical control indeed is possible. In the proof-of-principle experiment with teams around Ralf Röhlsberger (DESY, Hamburg) and Thomas Pfeifer (MPIK) at the Nuclear Resonance Beamline (ID18) at ESRF, we used the first part of the double-pulse sequence to excite an exciton within the nuclei, i.e., a single excitation coherently spread across many nuclei. The relative phase between the two parts of the double pulse then decides, how the second pulse continues the dynamics of the nuclear exciton. In particular, in the experiment, we demonstrated control over basic light-matter interactions between x-rays and nuclei, by switching between stimulated emission of the nuclear exciton, and further coherent excitation of the nuclei (see Fig. 8). These experimental results became possible using a powerful two-dimensional detection technique, which records the intensity of the x-ray light as function of time and energy. This multidimensional dataset contains rich interference patterns, which encode full holographic information of the complex-valued scattered x-ray field amplitude, allowing for the determination of the time-dependent complex dipole moment induced in the target nuclei. Our further analysis showed that the pulse shape control is exceptionally stable, with a temporal control stability of only a few zeptoseconds, opening perspectives such as multidimensional nuclear spectroscopy or nuclear pump-probe spectroscopy.
A key challenge in x-ray science is the characterization of spatial and temporal correlations on small scales. A promising method is time-domain interferometry (TDI), which promises access to correlations essentially background-free and over longer time scales than competing methods. In TDI, Mössbauer nuclei are used to split an incident x-ray pulse into two temporally separated parts. This double pulse probes the target system, which does not have to contain Mössbauer nuclei, at two different times. After the interaction, the two parts are overlapped in time, again using Mössbauer nuclei. The interference observed in the time-dependent intensity of the overlapped pulses then provides access to the correlations in the target. So far, TDI had only been analyzed for classical target systems and their classical correlations. However, quantum effects are expected to play a major role in strongly correlated and quantum materials, in and out of equilibrium. We therefore developed a TDI scheme that allows us to explore quantum dynamical correlations within the target, and to exclude classical models for the target dynamics. Quantum-TDI differs from the classical version by an additional phase control between the two interfering pathways, which can be realized by piezo-movements of the split unit. The quantum mechanical treatment shows that in TDI, a single photon is split into a time-bin entangled state, which subsequently provides information about the system at two different times, even though each realization of the experiment features only one interaction with the target. As a result, TDI allows for back-action free measurements of quantum mechanical correlation functions.
Extremely Intense Laser and High-energy Lepton Beam Interactions
Recent sustained technological progress such as the construction of the Extreme Light Infrastructure (ELI) is opening up the possibility of employing intense laser radiation to trigger or substantially influence physical processes beyond atomic-physics energy scales. Various topics in our division are devoted to laser-driven relativistic quantum dynamics and investigations of high-energy processes within the realm of quantum electrodynamics, nuclear and particle physics in extremely intense laser fields and high-energy lepton beams.
Quantum dynamics in intense laser fields
Quantum effects such as wave packet interference, entanglement, spin oscillations, and tunneling may be relevant when extremely intense laser pulses are impinging on atomic systems. In particular, a quantum tunneling barrier may be built up in an atom or a highly charged ion by the attractive Coulomb forces that attach the electron to the atomic core and the electric field of a strong laser that pulls the electron away from the core. Moreover, ultra-strong lasers can no longer be treated as pure electric fields; the laser's magnetic field component has to be taken into account, too (nondipole effects). The recent results of the group around Karen Hatsagortsyan contributed to an understanding of the role of sub-barrier as well as nondipole dynamics for shaping subtle features of the photoelectron momentum distribution in tunneling ionization.
One typical topic of interest is the fully relativistic time-resolved understanding of quantum processes during tunnel ionization of an atom in a very strong field. A simple model of this process claims that the electron tunnels instantaneously through the laser-generated quantum barrier and appears at its exit with vanishing momentum. However, the accurate description of the sub-barrier dynamics can significantly modify this simple picture. Our recent studies have identified a new pathway of strong-laser-field-induced ionization of an atom (see Fig. 9), which takes place via recollisions under the tunneling barrier. The interference of the direct and the under-the-barrier recolliding quantum orbits is shown to induce a measurable shift of the peak of the photoelectron momentum distribution which can be associated with a time delay. Based on a simple model without critical approximations, we showed that other conflicting approaches for the tunneling time delay, as e.g., the backpropagation method, can be reconciled with our sub-barrier recollision theory. For an experimental implementation a high resolution of momentum detection, better than 0.01 atomic units, is required to observe these signatures. One key observable is here the time delay in the so-called attoclock. We collaborated on this issue with the local experimental groups around Thomas Pfeifer and Robert Moshammer which together with our theory provided first experimental evidence for a nonvanishing Wigner quantum tunneling time.
We note that our concept of interfering under-the-barrier rrecollisions is extendable into the nonadiabatic regime and emphasize the two faces of the concept of the tunneling time delay. While the asymptotic time delay (ATD) is read out from the asymptotic photoelectron momentum distribution, the near-exit time delay (ETD) is considered theoretically and is observable in a Gedanken experiment with a so-called virtual detector near the tunnel exit (ETD). The peak of the tunneling wave packet emerging from the barrier around the tunnel exit significantly deviates from the most probable classical backpropagated trajectory, featuring a positive ETD. It however asymptotically merges with the backpropagated trajectory, which itself shows negative ATD, originating from the interference of the direct and recolliding sub-barrier paths. Finally, in explaining the missing detection of tunneling times via some other theoretical and experimental treatments, we have clarified that there are indeed no conflicts among the various approaches.
The nondipole description of the sub-barrier dynamics is also very important because it yields a nonzero momentum of the electron along the laser propagation direction at the tunnel exit. This modifies the photon momentum sharing in strong-field ionization as confirmed by recent experiments of Reinhard Dörner in Frankfurt. Further studies revealed the counterintuitive nature of the Coulomb field of the atomic core for the nondipole sub-barrier dynamics. Despite its attractive nature, the sub-barrier Coulomb field increases the photoelectron nondipole momentum shift along the laser propagation direction. The scaling of the effect concerning the principal quantum number and angular momentum of the bound state indicate that the signature of Coulomb-induced sub-barrier effects can be identified in the asymptotic photoelectron momentum distribution via a comparative study of the field-dependent longitudinal momentum shift for different atomic species. In addition the nondipole strong-field ionization time delay in a molecule due to the finiteness of the light speed manifests itself in the longitudinal momentum distribution, featuring double-slit interference (see Fig. 10), which efficiently encodes the molecular structure and laser parameters. The delay depends essentially on the tunneling exit distribution rather than on the molecular bond length – with corresponding consequences for the interference patterns.
Furthermore, the role of the spin may become essential in intense laser-matter interaction where we have previously investigated its dynamics and back action as well as compared deviating semiclassical models. More recently we have explored the possibilities for an efficient creation of polarized electron, positron and gamma-photon beams employing ultrastrong laser fields. In radiative polarization the electron spin flip is preferable along the instantaneous magnetic field in the rest frame of the electron. Because of that in ultrastrong laser fields initially unpolarized electrons are mostly polarized transversely after the interaction, while more beneficial for high-energy physics would be longitudinal polarization. In our recent investigation with former group members from China the role of the electrons’ anomalous magnetic moment on the helicity transfer from a circularly polarized (CP) laser pulse to an ultrarelativistic electron beam for the nonlinear Compton scattering process has been analyzed. While previous studies neglecting the QED radiative corrections came to a conclusion that the helicity transfer from laser photons to electrons is virtually forbidden in the nonlinear Compton scattering process, a significant longitudinal polarization of electrons is obtained when the one-loop QED vertex correction to the anomalous contribution to the magnetic moment is accounted for. The latter demonstrates also a signature of QED radiative corrections to the electron polarization dynamics in ultrastrong laser fields.
QED and high-energy processes in strong laser fields
The success of QED in vacuum calls for testing this theory under more extreme conditions such as those provided by strong electromagnetic background fields. The typical fields scale of QED is provided by the so-called Schwinger or critical fields of QED: Ecr=1.3×1016 V/cm and Bcr=4.4×1013 G. The team led by Antonino Di Piazza investigates theoretically the possibility of testing QED by means of ultra-strong electromagnetic fields with a focus on ultra-intense laser beams in these areas, where processes effectively occur in the presence of strong background fields of the order of the critical ones (strong-field QED). A linearly polarized laser field with an amplitude of the order of Ecr, would have a peak intensity Icr of about 4.6×1029W/cm2. Present laser systems are able to produce electromagnetic fields of intensities of the order of 1022 W/cm2 and future facilities like the Extreme Light Insfrastructure (ELI) and the Exawatt Center for Extreme Light Studies (XCELS) aim at laser intensities of the order of or exceeding 1024 W/cm2. Although the intensity Icr seems to be out of reach in the near future, the experimental investigation of strong-field QED is still possible. In fact, if an ultra-relativistic electron with energy ε collides head-on with a strong laser beam with electric field amplitude E, the strong-field QED effects depend on the Lorentz-invariant parameter χ≈2(ε/mc2) (E/Ecr), where mc2 is the electron rest energy. Therefore, by employing ultra-relativistic electron beams and intense laser fields, it is possible to render the parameter χ of the order of unity or larger, enter the strong-field QED regime, and test the predictions of QED under such extreme conditions.
Systematic analytical methods to investigate theoretically strong-field QED processes in intense laser fields have relied on approximating the background field as a plane wave, which allows to solve exactly the corresponding Dirac equation. In particular, by working within this approximation, we have performed the challenging computation of the one-loop vertex correction, which was the last missing block among the one-loop radiative corrections in strong-field QED in an intense plane wave. Radiative corrections in a plane wave also depend on the laser parameters. In particular, one-particle reducible contributions to the mass operator and to the polarization operator have been shown to increase with the pulse intensity and duration such that for sufficiently strong and/or long pulses it is necessary to resum all these contributions. We have recently carried out this analysis by constructing the electron/positron and the photon states by solving analytically the Schwinger-Dyson integro-differential equation within the so-called locally-constant field approximation (LCFA). The resulting states feature damping exponentials, which corresponds to the fact that electrons/positrons and photons are not stable particles in a laser field as the electrons/positrons emit photons and the photons decay into electron-positron pairs. These states have been then employed to compute the probabilities of the two basic strong-field QED processes, nonlinear Compton scattering (NCS) and nonlinear Breit-Wheeler pair production (NBWPP), including the damping of the particles’ states.
Despite its usefulness for gaining insight into strong-field QED, the plane-wave model can only account for the laser focusing effects in time and not in space. In order to describe QED processes in the presence of strong background electromagnetic fields of more realistic spacetime structures also including tight laser focusing in space, we have determined analytically the electron wave functions in laser fields of virtually arbitrary spacetime structure in the ultrarelativistic regime and by employing the WKB approximation. We have shown that the Baier-Katkov semiclassical formulas of radiation and pair production can be derived by using the found states to compute the probabilities of these processes. This casts the Baier-Katkov operator method within a self-consistent theory, which allows to controls the applied approximations and to compute the probabilities of more complicated processes like trident pair production. As an additional application, we have opened a new research area, where the so-called flying focus laser beams are employed to investigate strong-field QED. Flying focus beams are laser beams whose focal region can be made to move arbitrarily along the propagation direction of the beam (see Fig. 11). We have shown, in particular, that such beams can unveil the role of the transverse formation length in the radiation of high-energy photons by ultrarelativistic electrons, can amplify radiation-reaction effects, and can be used, provided they feature a definite angular momentum, to confine transversally high-energy electron beams over distances of the order of millimeters.
The understanding of the structure of QED in the presence of strong background fields cannot be fully accomplished without a thorough investigation of radiative corrections. Among these we have studied the self-interaction of the electron with its own electromagnetic field (mass operator) and the temporary transformation of a photon into a virtual electron-positron pair (polarization operator). Both analyses have shown qualitatively new effects with respect to the vacuum case. On the one hand, the structure of the mass operator has indicated that, unlike in vacuum, the electron spin dynamics can be substantially altered by the electron self-field. On the other hand, the investigation of the polarization operator has even shown that in the presence of a strong laser beam, virtual electron-positron pairs can propagate along distances which exceed by orders of magnitude the typical microscopic QED lengths of the order of the Compton wavelength λC=3.9×10-11 cm and are comparable with the laser wavelength (of the order of micrometers for optical lasers). As a consequence, the electron and the positron can absorb a very large number of laser photons (of the order of a million for an optical laser of intensity 1022 W/cm2) and the corresponding energy can be potentially exploited to prime high-energy reactions once the virtual pair again annihilates (see Fig. 12). A thorough analysis shows that this process is the analogous in the high-energy domain of the well-known recollision processes in atomic and molecular physics responsible, for example, of high-harmonic generation. Thus, such high-energy recollision processes may represent a basic, key ingredient for a miniaturized version of a high-energy "vacuum" collider.
Recently the study of the so-called QED or electromagnetic cascades has attracted a large attention from the strong-field QED community. Such electromagnetic cascades are described by means of kinetic equations, which determine the evolution of the electron, positron and photon distribution functions. Such equations, however, had been derived in a phenomenological way, based on conservation principles and on the properties of the quantum processes NCS and NBWPP. In collaboration with Prof. J. Berges and his student G. Fauth we have recently investigated strong-field QED phenomena by employing non-equilibrium quantum field theory and the so-called two-particle-irreducible (2PI) formalism. In this way, we have been able to derive from first principles the kinetic equations governing the dynamics of an electromagnetic cascade, which gave us the possibility of identifying the approximations which are implicitly used in the current description of QED cascades as well as possible theoretical extensions.
The relation between the electromagnetic and the gravitational interactions has always fascinated theoretical physicists especially in view of the unification of the four fundamental forces. We have recently exploited our experience in strong-field QED to investigate the gravitational radiation emitted by electrons driven by ultra-intense laser beams. By generalizing results obtained in classical electrodynamics and in QED in vacuum, we have demonstrated that the probability amplitude of emitting a graviton by an electron in an arbitrary plane wave is proportional to the corresponding amplitude of NCS. We have also demonstrated that the proportionality constant is the same in classical electrodynamics and in vacuum QED unifying in this way the previous findings in the more general treatment of strong-field QED coupled with linear gravity.
Quantum plasmas, gamma rays and relativistic laboratory astrophysics with laser and beam scenarios
It has been realized that recent technological advances in laser and beam systems pave the way to create analogous physical conditions in earth-based laboratories as those in violent astrophysical events like supernova explosions and gamma-ray bursts. “Relativistic laboratory astrophysics” is one of the youngest branches of astrophysics and one of the most promising, as it allows to investigate in a controlled and repeatable way extreme processes, which otherwise could be studied only indirectly. As a prominent example, the dynamics of an electron in the presence of extremely strong background electromagnetic fields, such as around pulsars and magnetars, is still one of the most fundamental open questions in physics. Indeed, this problem does not have a broadly accepted theoretical solution, and to date, little experimental data exist. One of the most fundamental challenges is to understand, and describe theoretically, the self-interaction of the electron with the radiation emitted by the electron itself, the so-called radiation reaction. This problem becomes even more acute in the so-called supercritical QED regime where χ>>1. In this case the emitting particles experience fields largely exceeding the critical field of QED in their instantaneous rest frame. It was conjectured by V. I. Ritus and by N. B. Narozhny that when χ>>1 QED could even behave as a strongly-interacting theory as QCD. As a possible route to probe the supercritical QED regime in the laboratory, the groups around Antonino Di Piazza and Matteo Tamburini have theoretically studied the propagation of an ultra-relativistic electron beam inside a crystal and the head-on collision of shaped ultra-relativistic electrons beams, respectively. They have shown that channeling radiation by ultra-relativistic electrons with energies of the order of a few TeV on thin tungsten crystals or the head-on collision of properly shaped tens to hundreds GeV electron bunches allows one to test the predictions of QED well inside the supercritical QED regime by measuring the photon spectrum. In particular, the collision of shaped lepton beams enables the unique characteristics that essentially all probe beam electrons (1) experience nearly the same extremely strong and localized electromagnetic fields. This implies that particles emit on average a single photon due to the shortness of the interaction between the probe beam and the source beam fields (see Fig. 13 left panel). Otherwise, for long inhomogeneous field interactions multiple low energy photon emissions broaden and soften the emitted photon spectrum. (2) Experience at the moment of emission rest frame fields that exceed the critical field of QED by orders of magnitude. This extremely high field strength in the rest frame of the emitting particle induces a spin-flip transition with a single photon carrying away nearly all the emitting particle energy. On the one hand, this setup allows one to measure the high-energy peak in the photon spectrum, the amplitude of the peak being proportional to the value of χ at emission (see Fig. 13 right panel). This opens up precision studies of strong-field QED in the ultra-relativistic regime. On the other hand, the proposed setup provides decisive advantages for the realization of an efficient multi-TeV laserless gamma-gamma collider based on electron-electron collisions because it permits to simultaneously suppress lower energy photon emission and to increase the probability of almost complete lepton-to-photon energy transfer.
To date, quasineutral ultrarelativistic electron-positron jets with dimensions of the order of the skin depth were generated via electromagnetic cascade initiated by the propagation of an ultrarelativistic electron beam through a high-Z solid. Although these results are promising, the formation of a dense and extended electron-positron pair plasma, one of the main challenges for relativistic laboratory astrophysics, has not been realized yet. In fact, the clear emergence of collective phenomena requires that the relativistic skin depth is several times smaller than the electron-positron jet dimensions. Dense and extended electron-positron pair plasma could be obtained from photon conversion into pairs initiated by a source of extremely dense collimated and high-energy gamma-ray beams.
The group around Matteo Tamburini has demonstrated via analytic modeling and state-of-the-art three dimensional simulations that such solid-density gamma-ray beams can be efficiently generated in the interaction of high-energy high-current electron beams with thin aluminum foils (see Fig. 14). In a nutshell, a pulsed, ultrarelativistic electron beam crossing a sequence of thin aluminium foils both self-focuses, therefore increasing its density, and efficiently yields a collimated gamma-ray pulse with more photons per unit volume than electrons in a solid. This occurs because the strong electric and magnetic fields accompanying the ultrarelativistic electron beam are “back-reflected” as the beam crosses the foil surface. Similar to an electromagnetic wave colliding with a mirror, the reflected magnetic field is nearly the same as the beam magnetic field, whereas the reflected electric field has the same amplitude as that of the electron beam but opposite direction. As a result, at the foil surface, the total electric field acting on the beam is nearly zero, while the total magnetic field is nearly doubled. This strong azimuthal magnetic field focuses the electron beam radially, and consequently triggers collimated high-energy photon emission. Remarkably, after passage of 20 foils, more than 30% of the electron beam energy is converted to gamma rays.
The advent of petawatt-class lasers enables GeV electron beams in strong electromagnetic fields to be studied experimentally using all-optical systems. We have studied several promising configurations that explore previously untested regimes. A setup of a unique x-ray source is put forward by a team led by Karen Hatsagortsyan, employing a relativistic electron beam interacting with two counterpropagating laser pulses in the nonlinear few-photon regime. The electron beam co-propagates along the stronger laser wave, while the counterpropagating laser beam is comparatively weak. The electron motion features two typical frequencies separated by orders of magnitude because of the Doppler effect. As a result, in the emission spectrum the Doppler-shifted high frequency peak is accompanied with satellites. While the gross features of the spectrum are determined by the counterpropagating laser beam, the subtle features are governed by the second copropagating laser beam (see Fig. 15). Accordingly, the bandwidth of satellites scales with the smaller characteristic frequency, allowing for bright ultranarrow x-rays. In contrast to Compton scattering sources, the envisaged x-ray source exhibits an extremely narrow relative bandwidth of the order of 10−4 and the brilliance of the x rays can be an order of magnitude higher than that of a state-of-the-art Compton source. By tuning the laser intensities and the electron energy, one can realize either a single peak or a comblike x-ray source in the range of several hundreds of eV to tens of keV. The laser intensity and the electron energy in the suggested setup are rather moderate, rendering this scheme compact and tabletop size, as opposed to an x-ray free-electron laser and synchrotron infrastructures. This radiation source is attractive for several applications, for instance, for resonant excitation spectroscopy of highly charged ions, for seeding of x-ray free-electron laser, or for extension of plasma diagnostics from the optical and UV range to the hard x-ray domain. Finally, replacing the laser fields with strong THz ones, the presented idea can be extended to a hard x-ray frequency comb for ultrahigh precision metrology.
We also put forward new methods for the plasma imaging via polarization properties of ejected particles. Understanding and interpreting the dynamics of ultrarelativistic plasma is a challenge, which calls for the development of methods for in situ probing the plasma dynamical characteristics. The ejected electron spin provides a new degree of freedom to extract information on the structure and magnitude of different components of the transient plasma fields, in particular, during the interaction of an ultrastrong short laser pulse with nonprepolarized near-critical density plasma in an ultrarelativistic regime. The particle-in-cell simulations show explicit correlations between the angle-resolved electron polarization and the structure and properties of the transient quasistatic plasma magnetic field (see Fig. 16). One can also employ for plasma diagnostics the polarization properties of the γ -photons emitted from a non-prepolarized plasma irradiated by a circularly polarized pulse. The angular pattern of γ-photon linear polarization is shown to be explicitly correlated with the dynamics of the radiating electrons, which provides information on the laser-plasma interaction regime. This diagnostics is especially advantageous for energetic and overdense plasma, and alike the generated x-ray pulses of interest for laboratory astrophysical studies.