A different type of "source" - Iron nuclei among cosmic rays
October 2006
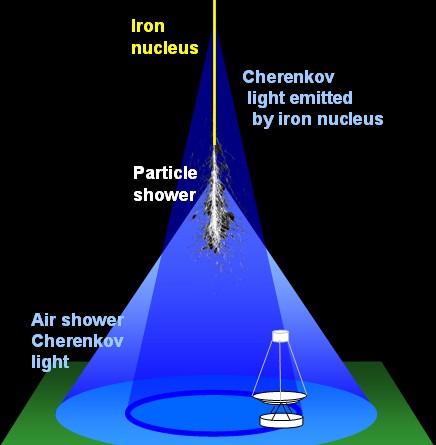
Sources and acceleration mechanisms of cosmic rays can be probed in two ways: either by imaging gamma rays (or neutrinos) generated when accelerated particles interact in or near the source, or by studying the cosmic rays arriving on Earth. This second method has the advantage that the cosmic rays are probed directly, but the disadvantage that all directional information is lost when charged cosmic rays are deflected by interstellar magnetic fields - that is, one cannot generate images using cosmic rays - and that spectrum and composition are modified when cosmic rays propagate through the Galaxy. The elemental composition of cosmic rays arriving on Earth has imbedded information about their origin (which elements are available to be injected into the accelerator? See e.g.Meyer et al. (1997)), about the acceleration mechanism (forces which act on the charge of a particle accelerate highly charged heavy nuclei more effectively than hydrogen nuclei, see e.g.Hörandel (2003) and references given there), and about the travel in space (the rate of certain isotopes among cosmic rays allows to estimate their residence time in the Galaxy, see e.g.Simpson (1983)). In particular at very high energies, measuring cosmic ray composition is a challenging task. Satellite- or balloon-borne instruments suffer from their small areas and cannot catch enough high-energy cosmic-rays. Ground-based air shower arrays, on the other hand, measure only the few secondary shower particles arriving at ground level and identifying the exact nature of the primary is difficult (e.g.Antoni et al. 2005). Cherenkov telescopes are normally used to detect gamma rays, butKieda et al. (2001) suggested a technique how such instruments can help to explore cosmic-ray composition in the PeV energy range: their idea is to detect the Cherenkov light emitted by the primary heavy nucleus high up in the atmosphere (see top figure). For most primaries, this "direct Cherenkov light" is hidden underneath the much more intense light from the air shower. However, Cherenkov light intensity grows with the square of the charge of a particle, resulting in an almost 700-fold enhancement for an iron nucleus (charge Z=26). In addition, since it is radiated at great height where the air is thin and the emission angle small, the direct Cherenkov light is more collimated than the light from the air shower. Now, H.E.S.S. succeeded for the first time to detect this trace of the primary cosmic-ray particle on the ground; while not a "gamma ray source", the result nevertheless seems worth to be featured in the "Source of the Month".
In total, 357 hours of H.E.S.S. data taken between 2004 and 2006 at small zenith angles were searched for the characteristic signature of direct Cherenkov emission from heavy nuclei: a concentrated very bright light spot at the head end of the extended shower image. Among these data, 1899 events were selected with the appropriate signature detected simultaneously in at least two telescopes. Fig. 1 shows a typical "direct Cherenkov" event: a single very bright image pixel - containing many 100 photoelectrons - is detected in close angular proximity to the reconstructed axis of the air shower, followed by a broad trail of air shower emission pointing away from the shower axis. As predicted, such events are only detected when the telescope is relatively close to the trajectory of the primary particle - about 70 to 120 m. A question is, of course, if such a signature could be faked by statistical fluctuations in normal images. On the basis of simulations, one expects about 10 background events per hour when the "direct Cherenkov light" signature is requested in a single telescope, already well below the detected rate of about 100 such events each hour. Final confirmation comes from events where the primary particle is detected simultaneously in 2, 3 or even 4 telescopes (Fig. 2); there, backgrounds are predicted to be completely negligible. As mentioned above, the H.E.S.S. analysis is based on the 1899 events where the primary nucleus is seen in at least two telescopes. Since such coincidences can only be seen when all telescopes happen to be at the right distance from the trajectory of the cosmic ray, the detection rate drops by about a factor 10 for each additional telescope. The charge resolution of the technique is not perfect, but is sufficient to identity and separate iron-like elements among cosmic rays and to determine their flux (Fig. 3). The new measurement is consistent with previous results and is more precise. The measured spectral index for iron nuclei in the 10 to 100 TeV energy range - 2.7 to 2.8 - agrees with the index of the overall cosmic ray spectrum, indicating that in this range the fraction of heavy elements among cosmic rays is roughly constant. Limited statistics currently prevent extension of the spectrum into the "knee" region where a change in composition is expected. As discussed byKieda et al. (2001), a dedicated instrument with even finer image pixels and nanosecond temporal resolution could further improve the charge reconstruction. However, the H.E.S.S. results show that the technique is not entirely free of systematic uncertainties related to the shower development: heavy fragments created in the first interaction of the nucleus contribute at reduced level to the "direct" Cherenkov light and the predicted rate of such fragments varies between different air shower models (Fig. 3).
References:
First ground-based measurement of atmospheric Cherenkov light from cosmic rays,H.E.S.S. collaboration, F. Aharonian et al., submitted for publication
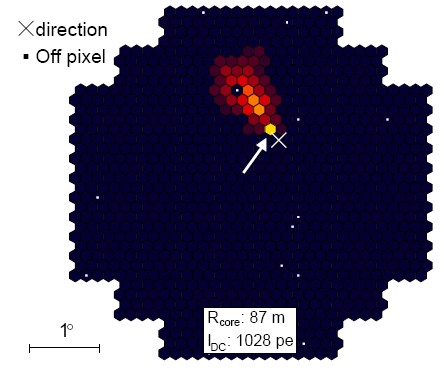
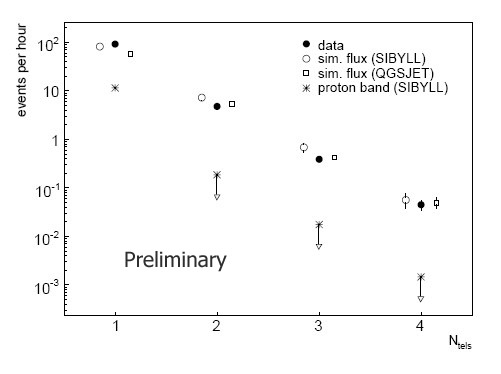
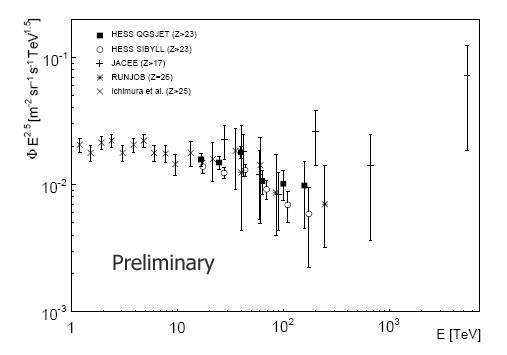