Research: History
Earlier Neutrino Physics Projects at MPIK
1) Borexino - Real-time solar neutrino spectroscopy at low energies
Solar neutrinos and Borexino's role
Figure 1: pp chain.
The nuclear fusion of four hydrogen atoms to one helium nucleus is responsible for the immense energy release from our Sun over billions of years. The Standard Solar Model (SSM) predicts that this overall fusion process occurs mainly via the so-called pp chain (98%), part of which is depicted in Figure 1. In the residual cases the energy is emitted in the catalytic CNO cycle. In stars with higher internal temperatures and higher masses the CNO cycle becomes dominant. That is the reason why the astrophysics community is also very interested in exploring this production channel in our Sun. Due to conservation of fundamental physics laws the fusion reactions do not only release a high energy of 26 Mega-electronvolts (MeV) in terms of light, but also 2 positrons and 2 neutrinos. Neutrinos are electrically neutral elementary particles, which exist in 3 flavors: electron, muon and tau neutrino. In case of the Sun, all released neutrinos are of the electron type. The weak interaction with matter allows neutrinos to escape the solar interior at almost speed of light and preserving the information about their origin. So, neutrinos are ideal probes for testing predictions of solar models.
Observations with all past and current solar neutrino detectors have shown a significant deficit in the measured electron neutrino flux compared to the theoretical predictions of solar models ('Solar Neutrino Problem'). This deficit was finally explained by a partial flip of the electron neutrinos into other neutrino types ('neutrino oscillations'), both during the propagation through the interstellar vacuum as well as enhanced by the presence of the high electron density inside the Sun ('Mikheyev-Smirnov-Wolfenstein (MSW) effect'). This discovery proofed the massive character of neutrinos, which were predicted to be massless by the standard paradigm of elementary particle physics. As a consequence the interest for searching new physics beyond it increased rapidly over the last two decades. From experimental point of view, solar neutrino experiments have continued to help defining the neutrino mass properties. The predicted solar neutrino spectrum covers a wide range up to 18 MeV, however 99% of solar neutrinos have endpoint energies E below 2 MeV: pp neutrinos have E < 0.42 MeV,
7Be neutrinos E = 0.38 MeV and E = 0.86 MeV, pep neutrinos E = 1.44 MeV, and the CNO neutrinos have E < 1.73 MeV. Comparing to all other previous and still running solar neutrino experiments, Borexino is the first and unique experiment world-wide able to detect several of these components individually, in real-time and with high statistics.
The Borexino Detector
Borexino detects neutrinos via their elastic scattering with electrons in the organic molecules of the scintillator. The detection of the expected number of solar neutrino interactions (few events for pep and CNO, few tens of events for
7Be, and about 150 events for pp) per day per 100 tons of scintillator sets stringent requirements to the allowed background levels due to cosmic-radiation as well as environmental natural radioactivity.
For these reasons, the Borexino detector has a graded shielding profile and was built in the National Underground Laboratory at Gran Sasso (LNGS) of INFN after several years of R&D (more info on the history of Borexino can be found
here). The overburden of the Gran Sasso dolomite massiv is 1.4 km thick. Thanks to it the muon flux is reduced by 6 orders of magnitude to about 1.2 muons/h/m
2. In order to further reduce the residual cosmic-ray flux the Borexino Inner Detector (see Figure 2) was surrounded by an Outer Detector. It is a domed steel tank with 16.9 m of height and 18.0 m diameter which is equipped with 208 photomultiplier tubes (PMTs) and filled with 2100 tons of ultra-pure deionized water. It allows to detect Cherenkov light generated by the residual cosmic particles and, moreover, to moderate and absorb neutrons emitted from the rock.
Figure 2: Borexino setup.
The Inner Detector contains organic liquids in three different subvolumes which are separated by two transparent nylon vessels. The two outer-most shells contain 890 tons (1350 m
3) of pseudocumene (PC) with few grams/l of the light quencher DMP. These buffer vessels act as absorber of external radiation and as Radon barriers. Within the inner-most nylon balloon (100 micrometers thick, 8.5 m in diameter) 278 tons (315 m
3) of an ultra radiopure scintillator constitute the core of the experimental apparatus. The scintillator is a mixture of pseudocumene (PC) and 1.5 g/l of PPO (a fluorescent molecule). The light induced by incident neutrinos as well as background events in the scintillator is viewed by 2212 PMTs which are mounted on the inner surface of the SSS and are pointing towards the detector's center.
The graded-shield of progressively lower intrinsic radioactivity towards the center culminates in powerful background identification and rejection techniques applied offline on the collected data. For instance, the ability to reconstruct the position of events, allows to apply a virtual space cut and use only the most internal 100 tons fo the scintillator ('Fiducial Volume') while the surrounding 200 tons are used as additional shield. Moreover, the reconstruction of the position and energy of events and their time stamp allows to search for coincidences induced by contaminants (e.g. Bi-Po coincidences from U/Th decay chain, or the cosmogenic radioisotopes
11C that can be tagged and rejected via a threefold-coincidence (TFC) method). Finally, pulse shape discrimination techniques have been successfully developed to reject e.g. alpha particles or to distinguish decays emitting either positrons or electrons.
Physics Program: Results and Future Prospects
Since the Borexino Collaboration widely succeeded to fulfill the radiopurity requirements, a rich physics program has been addressed, spanning from solar and geo-neutrino detection to the search for supernova and sterile neutrinos. A brief overview is given below.
Solar Neutrinos
7Be neutrinos
Figure 3: 7Be Neutrinos.
The main goal of the Borexino experiment has been the precise measurement of the monoenergetic
7Be neutrino rate. The signature of these neutrinos is a Compton-like edge at an energy of 0.7 MeV. The most recent evaluation includes 3 years of data-collection and its spectrum is shown in Figure 3;
7Be neutrinos and the main background components are free parameters, while the contribution of solar pp, pep and CNO neutrinos were fixed. The measured rate of (46±1.5(stat)±1.5 (sys)) counts/day/100 ton is in well agreement with the expectations of the SSM including the neutrino oscillations scenario. Recently, the Borexino collaboration studied the annual modulation of the solar neutrino flux due to Earth's eccentricity by means of the
7Be neutrino rate. A difference of 7% was expected. The absence of a modulation was rejected with high significance (more than 3 sigma).
8B Neutrinos
Figure 4: 8B Neutrino Spectrum
Borexino succeeded also to measure the 8B neutrino rate with an unprecedented low energy threshold of 3.0 MeV which is right above the highest energetic gamma-rays from natural radioactivity. The measured rate of (0.22±0.04(stat)±0.1(sys)) counts/day/100 ton is in good agreement with measurements from the previous solar neutrino experiments SNO and SuperKamiokande. These two pioneering experiments used successfully large Water Cherenkov detectors to measure solar neutrino interactions, however the energy threshold in those cases was only around 4.5 MeV.
pep neutrinos
Figure 5: pep neutrinos
The main obstacle for the detection of solar pep neutrinos in Borexino are radioactive decays of muon-induced
11C atoms. Their rate is 10 times higher than the expected underlying neutrino signal. Two powerful techniques were developed to identify and reject this background. The first method is based on a threefold-coincidence (TFC): In about 95% of cases
11C is produced by cosmic-rays (muons and muon-induced hadronic showers) through the reaction: muon +
12C → muon +
11C + n. The kicked-off neutron is absorbed in the scintillator shortly after (capture time: 255 microseconds) under emission of a characteristic 2.2 MeV gamma-ray. The
11C nuclide decays with a lifetime of 30 minutes into
11B plus a positron (e
+, antiparticle of the electron) and an electron-neutrino. The coincidence among muon, neutron and
11C decay allows to tag
11C candidates and to apply efficiently space cuts inside the Fiducial Volume for well-defined time periods. On this way, it is possible to reduce the
11C background by 90% while loosing only 50% of the collected statistics (upper plot in Figure 5). The second method looks for small differences in the pulse shape of events induced by positron (such as
11C) and electron emitters (such as neutrino-induced interactions). The technique allowed to reject most of the residual
11C background (lower plot in Figure 5). In this way, Borexino was able to measure for the first time directly the pep rate, namely (3.1±0.6(stat)±0.3(sys)) counts/day/100 ton.
pp neutrinos
Figure 6: pp neutrinos
The most recent analysis focused on the first direct detection of pp neutrinos which constitute almost 98% of the entire solar neutrino flux. The pp neutrinos have an endpoint energy of 0.42 MeV. Two main requirements for measuring them had to be fulfilled. First, the
85Kr continuous background had to be reduced. This was achieved after an extensive purification of the scintillator lasting more than one year. Second, the background induced by
14C decays had to be characterized and quantified.
14C is an intrinsic contaminant in the organic liquid mixure with endpoint energy at 0.25 MeV, which cannot be removed by any purification method. Since its trigger rate is so high, it often leads to pile-up events, whose spectral shape is a priori not known. Against expectations, Borexino succeeded to deduce the spectral shape of the
14C beta spectrum and its pile-up and finally to measure the pp neutrinos. The rate of (144±13) counts/day/100 ton is in good agreement with expectations of the SSM and neutrino oscillations. Moreover, the neutrino rate can be used to infer the expected solar luminosity. Because neutrinos can cross the Sun within seconds and photons produced in the Sun’s core take a very long time (at least several 10,000 years) to reach the surface, neutrino and optical observations in combination provide experimental confirmation that the Sun has been in thermodynamic equilibrium over such a timescale.
Geo- and reactor-antineutrinos
Figure 7: Geo-neutrinos
The so-called Bulk-Silikate Earth (BSE) model predicts the origin and size of radiogenic heat released in the Earth's interior and which coincides with the averaged value at surface by integrating over worldwide 40,000 deep bore-holes measurements. The heat is generated by decays of mainly U/Th. These decays are accompanied by a large number of anti-neutrinos (called 'geo-neutrinos') which can be detected by Borexino via the coincident inverse beta decay reaction: ν + p → n + e
+. On the other hand, cosmic-ray induced backgrounds, anti-neutrinos from European reactor plants and U/Th daughter nuclides (mainly
210Po) persist and have to be rejected. Borexino succeeded in this and measured (14.3±4.4) cts/100 ton within 1.7 years of life time. The value is in agreement with predictions of many BSE sub-models. In addition, the measured energy spectrum (Figure 7) allowed for the first time to separate the U from the Th content.
Future prospects in 2015 and beyond
Solar CNO neutrinos
The remaining neutrino flux among the 'Big Five' that has not yet been directly detected by any experiment is the one from the CNO cycle. So far, Borexino set the most stringent limit, i.e. < 7.9 counts/day/100 ton which is still 1.5 times higher than the SSM prediction.
At the moment of writing, the Borexino collaboration is attempting to determine directly the CNO neutrino rate. Besides the already mentioned
11C background, the major obstacle for the measurement is given by the unknown rate of
210Bi decays. Unfortunately, the concentration of the mother nuclid
210Pb is not in secular equilibrium with the decay product of
210Bi, namely
210Po. New quantities of
210Po atoms are often reintroduced in the scintillator during operations on the liquids. Several methods are under development to characterize the change in time of these tracers.
It's worth to mention that the measurement of the CNO neutrino rate will not only be useful for extrapolation to the models of other stars, but also to solve a more recent mismatch between the updated SSM and helioseismology predictions ('Metallicity problem'). Since the CNO neutrino flux would be strongly affected (29–40%) by the latest modifications of the SSM, a precise measurement will help to solve the problem.
Sterile neutrino search
Figure 8: 51Cr sterile neutrino signature in BX
Anomalies observed in several ground-based neutrino-beam experiments (LNSD, MiniBooNE) as well as deficits in anti-neutrino fluxes at short distances (10 m) measured in gallium and reactor experiments have suggested the existence of a fourth type of neutrino called 'sterile neutrino'. To confirm/rule out the hypothesis of such a new elementary particle a neutrino source experiment with Borexino is planned. The project called Short-distance Oscillation in BoreXino (SOX) forsees the deployment of high activity artificial neutrino (
51Cr) and antineutrino (
144Ce) generators externally to the Borexino detector at 8.25 m from its center. The expected oscillation pattern of the new type of neutrino in this configuration for the
51Cr devise is shown in Figure 8.
Supernova neutrinos and gamma-ray burst signals
Stars collapsing at the end of their life – so-called 'supernovae (SN) – release large amounts of light and neutrinos/antineutrinos. Only 3–4 supernovae per century are expected within our Galaxy. Since neutrinos travel at almost speed of light, and since the light emitted by the supernova is delayed by crossing the expanding stellar remnants, SN neutrinos typically reach the Earth earlier than the light. Thus, the early detection of SN neutrinos can be used to alert light detecting telescopes. This will help to measure the entire light curves of such cosmic explosions in different spectral bands. For this reason, a world-wide early warning network (called SNEWS) of large volume neutrino detectors has been established, whom also Borexino belongs to for several years.
More recently, Borexino has considered to join a cooperation with other experiments, which will look for gamma-ray bursts in terms of high-energetic events and gravitational waves.
More details about the Borexino results can be found in
some selected publications.
Borexino at MPIK
The group at MPIK joined Borexino before the construction of the experiment in the late 1990. The group led previously the pioneering solar neutrino experiment GALLEX/GNO experiment and thus was able to transfer its expertise in low-background techniques to Borexino.
Extremely low concentrations of radioactive isotopes in the various detector components of Borexino were required, thus large surveys in finding radio-pure materials were mandatory. The Borexino group at MPIK largely contributed in this material selection process by means of highly sensitive gamma-ray spectrometers, but also by mass spectroscopy and Radon-emanation measurements. A special contribution was the successful finding of an almost 226Ra free nylon foil suitable for the construction of the Borexino inner vessel. This is in direct contact with the ultra-clean scintillator and thus was a very critical part of the detector. The MPIK group in Borexino was and is still responsible for the control and reduction of background caused by the decay of radioactive rare gas isotopes (222Rn, 85Kr, 39Ar) in the scintillator and adjacent detector components. Most efforts during mainly the commissioning phase were dedicated to quality assurance of the supply chain of low argon/krypton nitrogen (LAKN2: 0.01 ppm Ar, 0.02 ppt Kr, 8 μBq/m3 (STP) 222Rn).
A hardware component developed at MPIK is the calibration system for the outer water Cherenkov detector to identify cosmic ray muons and to reconstruct their tracks. This system allows an improved discrimination of in-situ produced muon induced isotopes (e.g. 11C, 10C, etc.) which is the main remaining backgrounds for solar pep- and CNO neutrinos. From May 2007 the Borexino group at MPIK participated in the data collection on-site, in the data analysis and detector calibration. The analysis efforts have focused on the solar pep and CNO neutrino analysis. This includes the co-development and application of rejection techniques aiming at reducing the main background: the cosmogenic radioisotope 11C. For the description of a secondary background given by external gamma-rays from 228Th and 226Ra, the MPIK group developed a custom-made 5 MBq 228Th source and used it for two external calibration tests.
A list of Diploma and Ph.D. theses carried out at MPIK can be found
here.
The Borexino Collaboration
- Dipartimento di Fisica, Universitá degli Studi e INFN, Milano 20133, Italy
- Physics Department, Princeton University, Princeton, NJ 08544, USA
- Institut für Experimentalphysik, Universität Hamburg, Germany
- INFN Laboratori Nazionali del Gran Sasso, Assergi 67010, Italy
- Physics Department, Virginia Polytechnic Institute and State University, Blacksburg, VA 24061, USA
- Physics Department, University of Massachusetts, Amherst MA 01003, USA
- Lomonosov Moscow State University Skobeltsyn Institute of Nuclear Physics, Moscow 119234, Russia
- Department of Physics, University of Houston, Houston, TX 77204, USA
- St. Petersburg Nuclear Physics Institute, Gatchina 188350, Russia
- NRC Kurchatov Institute, Moscow 123182, Russia
- Joint Institute for Nuclear Research, Dubna 141980, Russia
- APC, Univ. Paris Diderot, CNRS/IN2P3, CEA/Irfu, Obs. de Paris, Sorbonne Paris Cité, France
- Physik Department, Technische Universität München, Garching 85747, Germany
- Kiev Institute for Nuclear Research, Kiev 06380, Ukraine
- National Nuclear Research University ”MEPhI”, 31 Kashirskoe Shosse, Moscow, Russia
- Max-Planck-Institut für Kernphysik, Saupfercheckweg 1, 69117 Heidelberg, Germany
- Dipartimento di Fisica, Universitá e INFN, Genova 16146, Italy
- M. Smoluchowski Institute of Physics, Jagiellonian University, Cracow, 30059, Poland
- Dipartimento di Chimica, Biologia e Biotecnologie, Universitá e INFN, Perugia 06123, Italy
- Istituto de Fisica Corpuscular, Valencia, E-46071, Spain
- Physics ans Astronomy Department, University of California Los Angeles (UCLA), Los Angeles, CA 90095, USA
The official webpage of the Borexino collaboration can be found
here.
Contact
-
Dr. Hardy Simgen:
Tel: +49 6221 516530
E-Mail: H.Simgen [at] mpi-hd.mpg.de
-
Dr. Werner Maneschg:
Tel: +49 6221 516287
E-Mail: W.Maneschg [at] mpi-hd.mpg.de
More Info
History and feasibility studies of the experiment
Figure 9: CTF
The history of the experiment starts in 1990, when a group of physicists envisioned a project aiming for measurements of low energy (below 2 MeV) solar neutrinos in real time. In order to get high light yield and thus a better energy resolution it was decided to use an organic liquid scintillator as detection medium. However, one had to cope with natural radioactivity (U, Th and noble gases
39Ar,
85Kr, and
222Rn) in the surrounding detector materials and in the scintillator. For the latter one the requirements were extremely high: 10
-16 g/g for U/Th and 10
-18 for
14C/
12C ratio. Since at that time it was not clear if these specifications can be fulfilled the collaboration decided to build a small-scale (4 tons active volume) pilot experiment called Counting Test Facility (CTF).
Figure 10: Borexino
Materials for the construction were carefully selected and delicate parts were mounted in a radon-free clean room. In 1995 CTF results showed the feasibility of the full-scale experiment, which was next approved by the agencies (in the years 1996–1998). In the time period of 2002–2004 the activity was stopped because of a scintillator spill accident. After restarting the operations, in spring 2006 the detector was filled with ultra-pure water, which was next replaced by approximately 300 tons of ultrapure scintillator. The filling finished on May 15, 2007, and the data collection was started immediately.
Back
Recent Borexino publications
-
Solar neutrinos:
- pp rate: Neutrinos from the primary proton–proton fusion process in the Sun, Nature 512 (2014), p. 383-386
- Summary of solar neutrino results from Phase I: G. Bellini et al., Final results of Borexino Phase-I on low energy solar neutrino spectroscopy, Phys. Rev. D 89 (2014) 112007
- 7Be rate @ 17%: C. Arpesella at al., First real time detection of 7Be solar neutrinos by Borexino, Phys. Lett. B 658 (2008) 101-108
- 7Be rate @ 10%: C. Arpesella at al., Direct measurement of the 7Be solar neutrino flux with 192 days of Borexino data, Phys. Rev. Lett. 101 (2008) 091302
- 7Be rate @ 5%: G. Bellini et al., Precision measurement of the 0.862 MeV 7Be solar neutrino interaction rate in Borexino, Phys. Rev. Lett. 107 (2011) 141302
- 7Be Day-night-asymmetry: G. Bellini at al., Absence of day-night asymmetry of 862 keV 7Be solar neutrino rate in Borexino and MSW oscillation parameters, Phys. Lett. B 707 (2012) 22-26
- pep rate & CNO limit: G. Bellini at al., First Evidence of pep Solar Neutrinos by Direct Detection in Borexino, Phys. Rev. Lett. 108 (2012) 051302
- 8B rate: G. Bellini at al., Measurement of the solar 8B neutrino rate with a liquid scintillator target and 3 MeV energy threshold in the Borexino detector, Phys. Rev. D 82 (2010) 033006
- Solar ν limits: G. Bellini at al., Study of solar and other unknown anti-neutrino fluxes with Borexino at LNGS, Phys. Lett. B 696 (2011) 191-196
-
Geo- and reactor-antineutrinos:
- G. Bellini et al., Observation of Geo-Neutrinos: Phys. Lett. B 687 (2010) 299-304
- G. Bellini et al., Measurement of geo-neutrinos from 1353 days of Borexino, arXiv: 1303.2571v1(hep-ex)
-
Muons and cosmogenic background:
- G. Bellini et al., Cosmic-muon flux and annual modulation in Borexino at 3800 m water-equivalent depth, Jour. Cosm. Astrop. Phys. JCAP05 (2012) 015
- G. Bellini et al., Muon and Cosmogenic Neutron Detection in Borexino: JINST 6 P05005 (2011)
-
Other rare processes:
- G. Bellini et al., New experimental limits on the Pauli forbidden transition in 12C nuclei obtained with 485 days of Borexino data, Phys. Rev. C, Vol. 81, No. 3, (2010)
- G. Bellini et al., Search for Solar Axions Produced in p(d, 3 He)A Reaction with Borexino Detector, Phys. Rev. D 85, 092003 (2012)
-
Detector and calibrations:
- G. Alimonti et al., The Borexino detector at the Laboratori Nazionali del Gran Sasso: NIM-A 600 (2009) 568-593
- H. Back et al., Borexino calibrations: Hardware, Methods, and Results: 2012 JINST 7 P10018
Diploma and Ph.D. theses carried out within the Borexino experiment at MPIK
- Werner Maneschg, Low-energy solar neutrino spectroscopy with Borexino: Towards the detection of the solar pep and CNO neutrino flux, Ph.D. thesis (2011), Karl-Ruprecht University Heidelberg
- Davide Franco, The Borexino Experiment: Test of the Purification Systems and Data Analysis in the Counting Test Facility, Ph.D. thesis (2004), Università Degli Studi di Milano and Karl-Ruprecht University Heidelberg
- Burkhard Freudiger, Untersuchungen zu den radioaktiven Edelgasnukliden als Untergrundquelle im Sonnenneutrinodetektor Borexino, Ph.D. thesis (2003), Karl-Ruprecht University Heidelberg
- Hardy Simgen, Hochempfindlicher Nachweis radioaktiver Edelgasnuklide und natürlicher Radionuklide aus der Uran-Zerfallsreihe, Ph.D. thesis (2003), Karl-Ruprecht University Heidelberg
- Johann P. Peiffer, Das Kalibrationssystem für den äußeren Detektor des Borexino Experiments, Diploma thesis (2003), Karl-Ruprecht University Heidelberg
- Grzegorz Zuzel, 226Ra in the nylon scintillator vessel as a background source in the Solar Neutrino Experiment BOREXINO, Ph.D. thesis (2002), Jagellonian University Kraków
- Christian Buck, Radonmessungen an Teilsystemen des Sonnenneutrinoexperiments BOREXINO, Diploma thesis (2001), Karl-Ruprecht University Heidelberg
- Wolfgang Rau, Low-Level-Radonmessungen für das Sonnenneutrinoexperiment BOREXINO, Ph.D. Thesis (1999), Karl-Ruprecht University Heidelberg
- Burkhard Freudiger, Bestimmung des Radon-Gehaltes in flüssigem Stickstoff, Diploma thesis (1998), Karl-Ruprecht University Heidelberg
- Hermino Neder, Ein Low-Level-Germanium-Spektrometer: Aufbau, erste Messungen und Simulationen, Diploma thesis (1998), Karl-Ruprecht University Heidelberg
- Matthias Laubenstein, Messungen von 226Rn und 226Ra im Rahmen der Counting Test Facility des Sonnenneutrinoexperiments BOREXINO, Ph.D. thesis (1996), Karl-Ruprecht University Heidelberg
Original motivation
The large amounts of electron antineutrinos produced in nuclear reactors provide information
related to the isotopic composition of the reactor core and its thermal power. There is an interest
in using antineutrino detectors as a potential safeguard tool. Therefore the studies within
the NUCIFER project [1] are dedicated to a large extent to safeguard and non-proliferation applications.
For example, the detection of a change in the antineutrino spectrum consistent with the removal of
a large quantity of 239Pu from the reactor would raise suspicions. The NUCIFER experiment was proposed
to IAEA (International Atomic Energy Agency) in October 2008 [2]. To allow that the antineutrino detector
can be placed very near to the reactor core (ca. 10 m) the detector should be compact, remote
controlled, safe and moveable. The detector is currently operated at the OSIRIS research reactor
at CEA-Saclay, France.
Do sterile neutrinos exist?
Recently a possible new application for this experiment of major scientific impact appeared. The
NUCIFER experiment could investigate an anomaly observed in the data of several reactor neutrino
experiments around the world. Measured values of the electron antineutrino flux appear to be only
94% of the value expected from theory [3]. So far, it is unknown whether this is due to unknown physics
that might involve weak mixing with a sterile neutrino or due to an overseen issue either related to
the experimental measurements or the theoretical flux calculations.
Detector design
The detector target is a 850 liter Gadolinium doped liquid scintillator filled into a cylindrical
stainless steel vessel. As in other reactor neutrino experiments as Double Chooz the antineutrinos
are detected via the inverse beta decay on Hydrogen nuclei of the target scintillator resulting in a
coincidence signal (separated by several microseconds) of a prompt (positron energy) and delayed
(neutron capture on Gadolinium isotopes) event. The scintillation light produced in the prompt and
delayed signal is detected by 16 photomultiplier tubes (PMTs) on top of the detector. Between the PMTs
and the target liquid there is a 25 cm thick acrylic buffer disk. Layers of polyethylene (15 cm) and
lead (10 cm) protect the target liquid against external radioactivity mainly originating from the
nearby research reactor. There is also an active veto made of 5 cm thick plastic scintillator panels.
The data acquisition system (DAQ) is build with commercial VME and NIM modules and it is operated with
a software interface written in LabView. Regular calibrations allow to monitor detector performance and
stability.
NUCIFER at the MPIK
MPIK provides the Gadolinium loaded organic target scintillator for the NUCIFER experiment. The main
challenge in developing metal loaded scintillator is to ensure long term stability of the optical
parameters as light yield and transparency. Similar methods as for the production of the Double Chooz
target scintillator [4] are applied and identical components are used. The concentrations of the individual
components were tuned to optimize background reduction in NUCIFER. In particular the target liquid was
tuned to allow for a pulse shape discrimination of fast neutron events, a main background source in the
experiment, and the coincidence time window is shortened due to an increased Gd-concentration.
Our group will also contribute to the analysis of the experiment, especially to learn more about the
reactor neutrino anomaly described above.
Future goals
In the future the NUCIFER detector might be used at a commercial reactor in France as well. Concerning
the search for sterile neutrinos new detectors are planned optimized for the needs of such an experiment.
The design and results of the NUCIFER experiment provide important input information for such
expermiments trying to solve the reactor neutrino anomaly.
References
[1] A. Porta for the NUCIFER collaboration, "Reactor Neutrino Detection for Non Proliferation with the
NUCIFER Experiment", IEEE proceedings, 10.1109/ANIMMA.2009.5503653 (2009).
[2] Focused Workshop on Antineutrino Detection for safeguard Applications, Final Report of IAEA Workshop,
IAEA Headquarters, Vienna (2008).
[3] G. Mention et al., Phys. Rev. D83, 073006 (2011).
[4] C. Aberle et al., JINST, 7, P06008 (2012).
Contact:
-
Dr. Christian Buck
Tel: 06221 516829
E-Mail: Christian.Buck@mpi-hd.mpg.de
- Prof. Dr. Manfred Lindner
Tel:06221 516800
E-Mail: manfred.lindner@mpi-hd.mpg.de
A main target in 20th century astrophysical research was the Sun. Understanding of the structure and the processes inside
the Sun is mandatory for any kind of star formation, galaxy formation or cosmological models. As the sun is the closest star,
it offers a unique approach for observation. Hans Bethe and some others
developed in 1938 a model, explaining the energy production in stars and especially inside the Sun by nuclear fusion process of protons.
Together with a model of the structure of the Sun and the conditions in its center (temperature, pressure, particle density etc.)
this reaction chain predicts an enormous flux of neutrinos, roughly 100 billion per second through a thumbnail, spread over a wide
range of energy (0.1 - 15 MeV).
Calculated energy spectrum of the solar neutrinos (with 1 standard deviation uncertainty) according to the
Standard Solar Model [1].
Ray Davis and coworkers were the first who tried to
observe solar neutrinos with a radiochemical detector. They used a chlorine solution as a target for inverse β-interactions:
νe + 37Cl → 37Ar + e.
The radioactive 37Ar could be extracted from the target and the decay could be detected with
proportional counters. The experiment of Davis had to face
fundamental problems: It was only sensitive to neutrinos with a energy higher than ∼0.8 MeV;
thus it could only detect neutrinos from the 7Be and 8B branch.
And, even more crucial, the observed neutrino flux was to low compared with the modell prediction. This discrepancy
lead to the so-called solar neutrino problem.
GALLEX: a radiochemical GALLium EXperiment to detect solar pp neutrinos
Until the year 1990 there was no observation of the initial reaction in the nuclear fusion chain.
This changed with the installation of the Gallium Experiments. Gallium as target allows neutrino interaction via
νe + 71Ga → 71Ge + e.
The threshold of this reaction is 233 keV, low enough to detect neutrinos from the
initial proton fusion chain. Low energy threshold is of great importance here since more than 98 % of the neutrinos
produced in the Sun are sub-MeV (pp: ∼91 %, 7Be: ∼7 %). Moreover the pp-neutrino flux is
coupled to the solar luminosity and therefore should be measured as precise as possible. Stringent limitations
of departures from the Standard Solar Model can be obtained if the flux of pp-neutrinos is deduced.
The target tank and the counting system was located in Hall A of the
LNGS.
The rock overburden shields the detector against interfering cosmic radiation.
This unique site is to find in central Italy in the Abruzzo region, close to L'Aquila at the highway A 24.
The highway tunnel through the highest mountain south of the Alps, the Gran Sasso (2912 m),
gives easy access to the worlds largest underground laboratory, with 3 large halls,
where several experiments were built in the last years.
The GALLEX was performed by the international collaboration with scientists from
France, Germany, Italy, Israel, Poland and the US, headed by MPIK Heidelberg. Spokesman of the collaboration
was the former head of the MPIK Neutrino Group, Prof.
Till Kirsten.
Outline of the experiment
In the time period of 1986 - 1990 the detector was constructed. The data taking started in May 1991. The first campaign
(GALLEX I) was finished in May 1992. The average result of the GALLEX I 15 solar runs was (83.4 ± 19) SNU [2].
It was announced for the first time at the "Neutrino 1992" conference in Granada on June 8th.
A transparency by T. Kirsten announcing the first observations of the pp neutrinos by GALLEX.
Neutrino 1992 conference, Granada, June 8th.
Until February 1997 (end of solar data taking) there were three more campaigns: GALLEX II, III and IV. During that
period the detector was calibrated twice using an artificial neutrino source (51Cr): in 1994 [3] and in 1995/1996 [4].
This was a very important demonstration of the reliability of the GALLEX detector and of the radiochemical method in general. At the
end of the experiment, in 1997, an arsenic test was also performed (using 71As). The goal was to check the influence of
the so called "hot chemistry" on the observed neutrino rates [5].
Recently the data form GALLEX (including the calibration tests) were re-evaluated using a full pulse shape
analysis instead of originnaly applied rise-time techniques [6].
The GALLEX detector and 71Ge extraction procedure
As already mentioned the GALLEX target consists of 30.3 t gallium in the form of a concentrated GaCl3-HCl solution
(total weight 101 t). For safety and redundancy reasons, GALLEX had two nearly identical target tanks (A and B).
Until April 1992 the solar neutrino measurements have been performed in the B tank (GALLEX-I). On April 30, 1992,
the GaCl3 solution was transferred to the A tank which is equipped with a central reentrant tube designed
to hold a 51Cr neutrino source (for future calibration experiments). After various tests were performed,
measurements of the solar neutrino flux were resumed in August 1992.
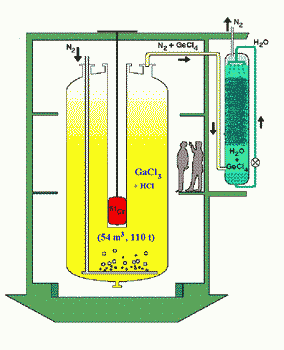
Scheme of the GALLEX detector tank with the absorber system and the Chromium source inserted inside the
thimble.
At the beginning of a new run (i.e. start of exposure) a carrier solution containing about 1 mg stable Ge carrier was added to
the GaCl3 solution. For this the non-radioactive isotopes 72Ge,74 Ge and 76Ge,
later also 70Ge, are sequentially used in order to monitor carryover from one run to the next one. After three or
up to weeks of exposure time, desorption starts by purging the target solution with 1900 m3 of nitrogen in 20 hours.
Because tank A is equipped with a more efficient sparger system, the desorption time there was reduced to 12 hours. Germanium
is removed from the solution as volatile germanium chloride (GeCl4). The gas stream was passed through a system of
water scrubbers where the GeCl4 was absorbed. At the end of desorption all the germanium was contained in a volume
of about 30 liters in the first scrubber. A series of smaller columns serves to further concentrate the germanium in a volume
of about 1 liter of water. The final concentration step is an extraction into CCl4 and a back-extraction
in to 50 ml of tritium-free water. This step also serves to separate the germanium from any tritium in the tank or the absorber
system, since tritium would contribute to the background in 71Ge counting. The last part of the chemical procedure
is the conversion of GeCl4 to the gas GeH4 by means of the reducing reagent sodium borohydride
(NaBH4). The GeH4 is dried and purified by gas chromatography . Its volume is then measured in order to
determine the overall yield of the chemical procedures. Finally, it is placed together with Xenon into a miniaturized proportional
counter. The volume ratio Xe:GeH4 is 70:30, the total counting gas pressure is about 800 Torr. The total chemical
yield (i.e. the ratio of the Ge amount extracted to the amount of Ge carrier added to the target solution at the beginning of the
run) was about 99 %.
Counting with miniaturized proportional counters.
The 71Ge decay rates expected in GALLEX even if the SSM signal would be observed are below 1 per day . The measurement
of such low decay rates is an extreme low-level task and could only be achieved with miniaturized proportional counters.
71Ge decays by K (87.7 %), L (10.3 %), and M (2.0 %) electron capture. Neglecting the M events, the energy deposition
from Auger electrons and X-rays emitted in the decay results in a energy spectrum with two peaks: an L peak at 1.2 keV and a K
peak at 10.4 keV. All provisions typical for low-level counting have to be applied in order to reach the extremely low
background rates required: for the proportional counters itself this implies miniaturization and the use of ultrapure (with respect
to radioactive contamination) materials. The counter body is made from Suprasil quartz. A special quartz blowing technique allows to
standardize the counter dimensions. The cathode is either made from zone-refined iron (Fe counters) or machined from a single Si
crystal (Si counters). The anode is a 13 μm tungsten wire. The active volume (volume inside the cathode) is typically 87 % of the
total gas volume (which is about 1 cm3).
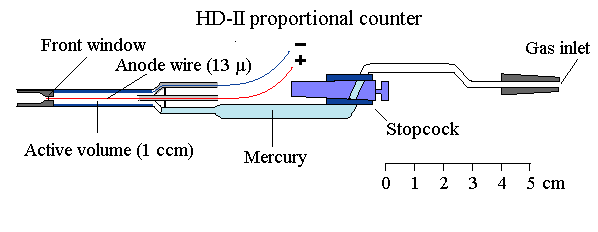
Miniaturized ultra-low background proportional counter HD-II type.
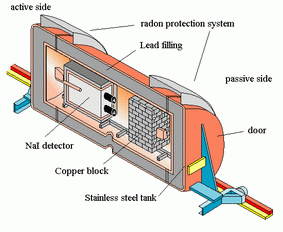
Scheme of the proportional counter shielding tank installed at the counting building inside a faraday cage.
The counters were placed into a copper box containing the preamplifier. The boxes are inserted either in one out of eight counting
positions in the well of a NaI pair spectrometer ("active shield/side") or in one out of sixteen positions within a massive copper block
("passive shield/side"). Both the NaI detector and the Cu block are installed inside a steel vessel filled with 8.6 tons of low radioactivity
lead. The shield can be opened by moving one of the two sliding end doors (see Picture above). Radon in the air
surrounding the counters can produce background counts by the gamma and beta radiation emitted in the decay of its daughter
nuclides 214Pb and 214Bi. In order to keep this background contribution as low as possible, the shielding
tank is air sealed by a glove box system installed around it. Counters were transferred in to the shield or removed from it by means of
an air lock. The radon content inside and outside the shield is continuously monitored by two Lucas chambers with 5 hours integration
time. While the radon content of the air outside the shield (depending on the venting situation in hall A) fluctuates between
∼50 and 100 Bq/m3 (in extreme cases 1000 Bq/m3 were reached) the level inside the shield is usually
below 1 Bq/m3 (detection limit for the used Lucas cell). From a calibration measurement we know that such a level amounts
to (0.0015 ± 0.0005) counts per day in the counting windows on the active side. On the passive side the effect is 20 times lower.
The counter shield together with the electronics was installed inside a Faraday cage.
In order to calibrate the energy and rise-time scale of the GALLEX counters, a 153Gd-Ce X-ray
source was used. The europium X-rays following the electron capture decay of 153Gd (T1/2 = 242 days)
excite the characteristic K alpha and K beta X-rays from a Cerium target. These are used to illuminate the entire proportional
counter volume rather homogeneously leading (in addition to the photopeak from the 35 and 40 keV X-rays) to three peaks
at energies of 1.03, 5.09 and 9.75 keV which result from the escape of Xe X-rays. Typical energy resolutions (FWHM) of the GALLEX counters
were 43 % for the L peak and 26 % for the K peak. Average absolute counting efficiencies were 28.6 % in the L window and 33.9 % in the K
window for the nine counters with Fe cathode used in the GALLEX I runs. The corresponding average value for the six counters with Si cathode
are 29.7 % (L) and 31.7 % (K).
Outcome of the experiment.
After four campaigns: GALLEX I - GALLEX IV, 65 solar runs and 1594 days of data taking the final GALLEX result was (77.5 ± 7.7) SNU
which should be compared with the expected 126 SNU according to the Standard Solar Model and no-oscillation scenario.
From the calibration tests with the 51Cr source the obtained ratios of measured to expected rates were (1.00 ± 0.11) and
(0.83 ± 0.10), respectively confirming the proper performance of the detector. Any Ge-yield errors above 1 % was excluded according
to the 71As test.
GALLEX provided the very first observation of the solar pp neutrinos and thus an important confirmation of the
nuclear fusion process inside the Sun. The measured neutrino signal was smaller than predicted by the Standard Solar Model (c.a. 60% of the
total expected flux has been measured), however the reduction factor was different then in the chlorine experiment. At that time two
explanations were possible: either the model is not correct, or the neutrino has some features which were not yet taken into account in
the Standard Model of elementary particles.
In 2007 the GALLEX data were re-evaluated using a full pulse shape analysis (instead of applied originally
rise-time technique). In addition all individual counters were calibrated what resulted in the calibration error reduction. Also radon cut
efficiency has been improved. After corrections the overall GALLEX result has slightly decreased down to (73.1 ± 7.2) SNU. As can be seen
in the Figure below among the four GALLEX data taking periods, by far the largest change is for GALLEX IV. This period had created some
concern because of a ±2 σ deviation from the mean, which is now reduced ±1 σ. Re-analysis of the 51Cr
experiments has been performed with new efficiencies and an improved solar subtraction (including also GNO data). This update has changed
the average result (ratio of the measured to expected neutrino flux) from (93 ± 8) % down to (88 ± 8)%. Taking also into account
the Cr-source results obtained by the SAGE collaboration one should consider
re-evaluation of the 71Ge production rate on Ga, which should be reduced by ∼2 SNU for 7Be neutrinos from
34.8 SNU down to 32.7 SNU (there is no change for pp neutrinos).
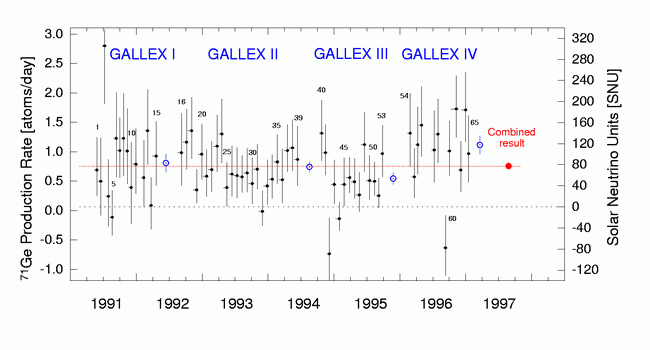
GALLEX single run result overview. The left hand scale is the measured 71Ge production rate; the
right hand scale, the net solar neutrino production rate (SNU) after subtraction of side reaction contributions.
Error bars are ± sigma statistical only. The label 'combined' applies to the mean global value for the total of all 65
runs. Horizontal bars represent run duration; their asymmetry reflects the 'mean age' of the 71Ge produced.
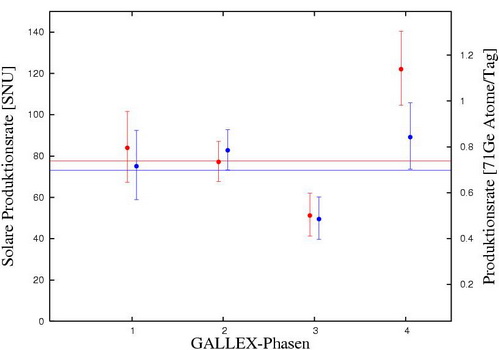
Re-evaluated GALLEX data (in red). The biggest change appeared for the GALLEX IV period. The overall result
is by 4.4 SNU lower than reported earlier.
GNO: the Gallium Neutrino Observatory.
GNO was the successor project of GALLEX, with scientists from Italy and Germany, now headed by the Milano group of the INFN.
Spokesman of the Collaboration was Prof. Enrico Bellotti (Milano, Italy).
GNO aimed to measure the flux of solar electron neutrinos from the proton-proton fusion during a full
solar cycle. A special goal of the collaboration was the reduction of systematic uncertainties in the result
of the observation (with respect to GALLEX), and, simply due to the prolonged time of observation, a smaller statistical error.
During 1997/98 big effort was set on an upgrade of the DAQ electronics and some modifications in the synthesis process.
It has been proposed to the scientific community and to the funding agencies to increase the mass of gallium up to 100 tons
(from 30.3 tons). The first solar runs started in April 1998 and the last one was performed in 2002. The experiment was officially
finished in 2004.
Main changes in the GNO experimental procedures as compared to GALLEX are the following [7]:
-
Desorption time and desorption gas volumes for GNO have been reduced from 12 to 9 hours and from 2500 to 1700 m3
(at 20 ◦C and 0.9 bar). This simplified the operating schedule at the expense of a slightly less efficient Ge desorption.
The un-desorbed Ge-isotope carrier fraction remaining in the tank was expected to increase from about 0.2 % to values between
1 % and 2 %. The fact that a homogeneously distributed Ge hold-back carrier level never drops below ∼10−13 mol per liter helps
to exclude hypothetical 71Ge loss scenarios that would involve the carrying of non-measurable ultra-low trace impurities
below that level.
-
The major contribution to the systematic errors of the GALLEX results (∼4 %) came from the insufficient knowledge of counter
efficiencies (3.5 %). This is due to the fact that efficiencies for counters used in solar runs have not been measured
directly because of contamination risk. Instead, they have indirectly been evaluated from measurements on other counters combined with
a scaling procedure based on Monte Carlo simulations. However, some of the inputs needed in these MC simulations (counter volume, gas
amplification curve) are not known with the accuracy that one can ambitiously desire. In order to decrease this systematic error
substantially, the GNO Collaboration has developed a method that allows direct counter efficiency calibrations without introducing a
major contamination risk. The resulting total errors came out between 0.8 % and 1.4 % (average 1.1 %) what constitutes a substantial
reduction, as anticipated.
-
An elaborate radon test was performed with a modified counter containing a 226Ra source. The aim was to improve the
characterization of radon events in the GNO proportional counters. The recordings lasted from May 1999 through March 2001 (1.8 years of
counting time). After this long-lasting measurement, the emanation valve was closed and the intrinsic background of the counter was
measured for 2.0 years. Using these data, we re-evaluated the inefficiency of the radon cut. The result is consistent with zero and the
(2 σ) upper value is 7.3 %. This replaces the formerly determined value used in GALLEX: (9 ± 5)%.
-
Analog and digital electronics, power supplies and data acquisition system have been completely renewed and reorganized after the
accomplishment of GALLEX data taking. The analog bandwidth of the system has been increased to 300 MHz, the typical RMS noise is 2.8 mV.
Due to these improvements and to a thorough screening of counters used in solar runs, the background in GNO is 0.06 counts per
day in the relevant windows, corresponding to a 40% background reduction compared to GALLEX.
-
In addition to solar runs, one-day-exposure blank runs were also performed regularly in order to verify the absence of any artifact or
systematics related to the target. During the period of operation of GNO, 12 blank runs were successfully performed.
The absence of spurious effects or unknown background is confirmed by the fact that the small
excess of 71Ge counts in the blanks is consistent with the neutrino-induced production rate during the short exposure and the
carry-over of the previous solar run.
-
For the selection of the 71Ge events a new neural network pulse shape analysis (NNPSA) and a subsequent maximum
likelihood analysis has been used instead of rise-time technique (GALLEX).
Outcome of the experiment.
The total GNO neutrino exposure time was 1687 days (58 runs). During this time, the maximum likelihood analysis
identified a total of 258 decaying 71Ge atoms (131 L, 127 K), 239 of them (or 4.1 per run) due to solar
neutrinos. The mean 71Ge count rate per run and counter at the start of counting is ∼0.27 counts per
day. This may be compared with the time independent counter background as low as ∼0.06 counts per day (average).
The individual run results for the net solar production rates of 71Ge (based on the counts in the K and
L energy- and neural network acceptance region, after subtraction of 4.55 SNU for side reactions and after corrections
for annual modulations) are plotted in Figure below. The combined net result for all GNO runs is 62.9+6.0-5.9 SNU
(1 σ, including systematics).
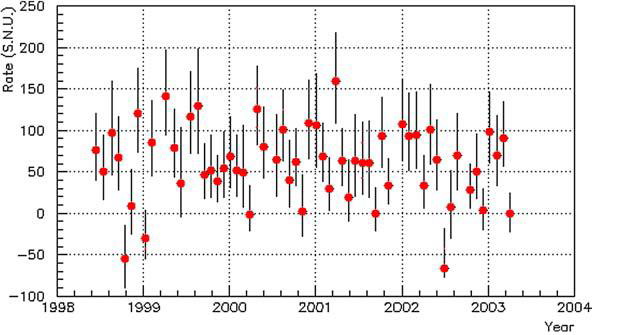
GNO single run results. Plotted is the net solar neutrino production rate in SNU after subtraction of
side reaction contributions. Error bars are ±1 σ, statistical only.
Combined analysis of the GALLEX and GNO data.
The Figure below shows single run results for GNO and GALLEX (before re-analysis) during a full solar cycle. Plotted is the net
solar neutrino production rate in SNU after subtraction of side reaction contributions. Error bars are ±1 σ, statistical
only.
The joined GNO + GALLEX result after 123 solar runs is 67.5 ± 5.1 SNU (1 σ, after GALLEX data reanalysis).
The joint result confirms the earlier GALLEX outcome which made the case to claim strong evidence for non-standard neutrino properties
because the production rate predictions from the various standard solar models have always been much higher (120 - 140 SNU) than the
measured rates. The updated result of the SAGE experiment, 66.9+5.3-5.0 SNU (1 σ), agrees well with the
GALLEX+GNO analysis.
The GALLEX and GNO data have been also analyzed relative to a correlation with the seasonal Earth-Sun
distance variation. The 123 solar runs have been divided into 6 about equally populated bins of similar heliocentric distance d.
The fit assuming a solar neutrino rate constant in time and affected only by the 1/d2 geometrical modulation yields a
confidence level of 69 % (χ2 = 3.0 with 5 d.o.f.). The difference between rates of the solar runs performed
in winter time W (defined as perihelion ±3 months) and in summer time S is δ(W −S)=−7.6 ± 8.4 SNU (the value expected
from the 1/d2 modulation only is +2.3 SNU). The finding that the solar data are consistent with a production rate constant
in time does not invalidate however other hypotheses that might give similar or even better (short) time dependent fits.
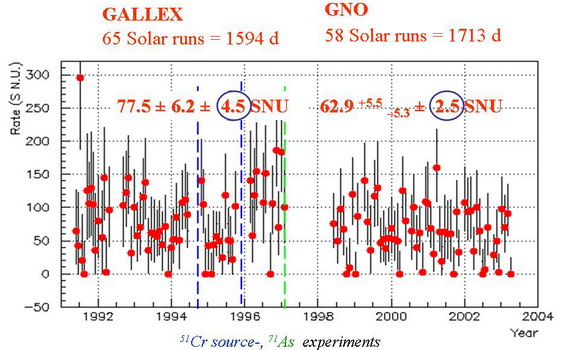
GALLEX and GNO data with marks indicating the calibration and arsenic tests.
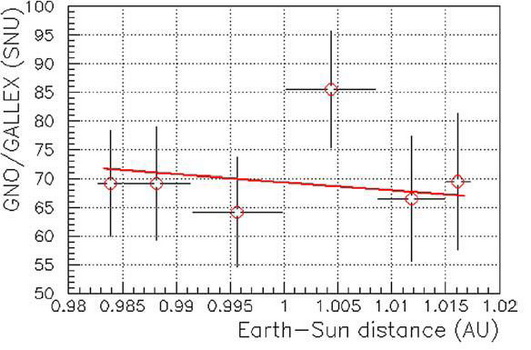
GNO/GALLEX signal vs. heliocentric distance of the Earth. The straight line indicates the expected flux
variation due to purely geometrical (1/d2) effects.
If the LMA (MSW) solution is the correct explanation of the SNO/SK data, then vacuum oscillations must dominate below 1 MeV and the
mixing angle is estimated as (32 ± 1.6) degrees. From the GALLEX (and GNO) data one can extract the survival
probability Pee for pp-neutrinos after subtraction of the 8B and 7Be contributions based on the experimentally
determined 8B- (SNO/SK) and 7Be- (Borexino) neutrino fluxes as Pee (pp only) = 0.52 ± 0.12. The results imply
the experimental verification of the solar model and of the neutrino oscillation mechanisms at sub-MeV energies that are otherwise
inaccessible.
Summary
Radiochemical experiments gave cumulative measurement of the integral solar neutrino interaction rate (as opposed to real-time event
detection, also there was no spectral or directional information). For the first time the low-energy pp-neutrinos have been registered
confirming the nuclear fusion process inside the Sun. After a long time of operation of these first generation detectors (Cl, Ga),
the statistical errors equaled the intrinsic systematic errors. The success of these experiments implied their end.
However, there were still reasons to continue observations of the low energy neutrinos (GNO):
-
Continuous pp-neutrino monitoring is an astrophysical necessity
-
pp-observations simultaneously with Borexino real-time beryllium neutrino observations
-
Further neutrino source experiments to improve the knowledge of relevant cross sections
References
[1] Bahcall, Serenelli and Basu, ApJ, 621, L85 (2005)
[2] GALLEX Collaboration PL B285 (1992) 376 and PL B285 (1992) 390
[3] GALLEX Collaboration PL B342 (1995) 440
[4] GALLEX Collaboration PL B420 (1998) 114
[5] GALLEX Collaboration PL B436 (1998) 158
[6] F.Keather, PhD Thesis, Heidelberg University, 2007
[7] GNO Collaboration PL B616 (2005) 174
Sources
[1] W. Hampel: Talk at the Royal Society, London, 1994. Published in "Phil. Trans. R. Soc. Lond. A (1994) 343, 3-13"
[2] T. Kirsten: Talk at the TAUP 2007 Conference, 11-15.09.2007 Sendai, Japan
People involved
The Heidelberg-Moscow-Experiment was a German-Russian Collaboration
between the Max-Planck-Institut für Kernphysik and the
Kurchatov Institute in Moscow, Russia. The experiment searched for
neutrinoless Double Beta decay of
76Ge.
The experiment was operated in the Gran Sasso Underground Laboratory
in Italy with five detectors with a total mass of 10.9kg of Germanium
which was enricht to 86% with the double beta emmitter
76Ge.
The Gran Sasso underground laboratory reduces the cosmogenic muon flux
by six orders of magnitude and further shielding was provided by lead
around the detectors. Finally, further background reduction was achieved
by Puls Shape Analysis (PSA) which allows to discriminate background
from signal events.
A sub group of the original collaboration claims as a final result for
the half life of neutrinoless double beta decay of
76Ge:
T = (2.23 +0.44 -0.31) x 1025 years (99.97% C.L.)
This positive result has been heavily debated amongst experts.
This result is even very interesting if a sigal can not be established,
since it is still the strongest limit on neutrinoless double beta decay
unti now. This has profound implications on physics beyond the Standard
Model, like supersymmetry, Left-Right Symmetry, Compositeness...
which will be tested at LHC.
People involved
- Prof. H.V. Klapdor-Klingrothaus
M-Cavern: Micro-Chemistry Analysis of Various Extra-terrestrial and environmentally found RadioNuclides.
Scientists from MPIK have gathered wide expertise in the field of low-background physics, working in meteorite and mineral
research, GALLEX/GNO, BOREXINO and LENS.
Measurement devices like Ge or NaI gamma spectrometers, proportional counters, scintillation cells, mass spectrometer etc.
are routinely used at LNGS and at the MPIK. Furthermore, the MPIK is equipped with an on-site
Low-Level-Laboratory, providing a shelter against
the hadronic constituents of cosmic rays.
In 2002 MPIK in Heidelberg, Germany and the Space Science Division, E. O. Hulbert Center for Space
Science of the US. Naval Research Laboratory (a laboratory involved in many aspects
of solar satellite research and space exploration)
created a cooperative research project according to a proposal of F. Hartmann located at Gran Sasso: MCavern [1].
The MCavern project combines mutual expertise to create a new low background counting facility for the development of
detectors and techniques. It was expected not only to be of use to the field of Particle Astrophysics, but to Fundamental
Chemistry and applications in Space, Atmospheric and Ocean Science, as well.
The project comprises, in the first stage, the construction of new ultra-low background germanium
detectors as well as proportional counters, a shielded counting setup, a gas and vacuum system, counter filling lines and some
already existing chemistry equipment. A sample handling area and small (sub-gram) chemistry units were proposed thereafter.
Ge-spectrometers: GeMPI II and III
In the frame of the project a new GeMPI-type [2] germanium spectrometer has been constructed at the LNGS (the next - GeMPI III is under
construction). The cutaway view of
the detector shows (see below) the sample entry air-lock system, glove box access holes, shield (copper and lead), specially
designed cryostat and liquid nitrogen dewar holding special charcoal absorbers. Samples pass through the air-lock, past a heavy
sliding lead copper door and into position just above the germanium crystal whose special housing of ultra-low background parts
is all fabricated by MPIK engineers and scientists. A specially constructed lead block made by INFN shields from the internal
electronic components within the crystal can.
Recorded background spectra show background level which is slightly lower than that observed for GeMPI I [2].
A schematic view of the GeMPI II detector with the shield and the Rn-box. The design was based on the GeMPI
detector operated by MPIK since 1998 and used for material screening in the frame of several projects like BOREXINO or LENS.
The gas counting project
The gas counting project was based on the development of a new instrumental capability:
1) to count multiple gases at the few event level, not tuned to just one specific gas
2) to separate and collect a number of different gas components from the same gas sample
3) to be able to analyze the gas content of liquids (e.g. scintillators)
4) to be able to synthesize counting gases from low level source materials
This system evolved from prior systems [3,4,5]. The basic design is that inherent in the approach
of Ray Davis. The first gas line used for few atom counting of Ar was an essential feature of the
Homestake Chlorine Experiment.
This type of line was later modified and extended to handle the specific needs of the Gallex Experiment by Brookhaven and MPIK.
The actual GALLEX lines transferred to the GNO Project (also upon which the Brookhaven group exited).
A goal since then was to extend the capability to other gases. During GALLEX it was found using a dedicated "Rn counter"
that few atoms of 222Rn could be processed in the GALLEX lines and counted. But the GALLEX lines as constructed
were not suited to be dedicated to this purpose. In addition to these synthesis lines, there was in GALLEX a counter filling
line. This line was later transported to Hall C at LNGS to support 222Rn measurements in the BOREXINO Project. This line is
primitive in construction and essentially comprised of discarded parts and systems.
In addition to the gases of 222Rn [6], the lines at Gran Sasso have seen limited scope handling of
133Xe, 135Xe counting as part of GALLEX tests [7]; and most significantly GALLEX studied the removal
of 71As also produced as a gas. The counting of 133Xe, 135Xe later became a sub-project
in the LENS arena. Some ideas to count gases from the BOREXINO scintillator (at first CO2 and 85Kr)
ran into difficulties because of the lack of a separate gas facility. Since then a counter filling line at Heidelberg was
modified to incorporate 85Kr counting and tests of this were quite good [8]. Even with the clearly limited
capability of use of the two modified counter filling lines (the one at Gran Sasso lacking gas chromatography) Heidelberg
has achieved remarkable success in contributing to low background measurement for the projects at Gran Sasso.
A diagram showing the proposed line structure and support system is shown in figure below. As mentioned
the design was based on the existing devices located in Heidelberg and at Gran Sasso.
Diagram of the Gas Handling, Chromatography and Counter Filling System. The system is built up around the
Chromosorb 102 column designed for efficient separation of gases with low 222Rn emanation as developed for Ar
(Homestake), Ge, Xe, Rn (GALLEX, BOREXINO) and tested for Kr by H. Simgen in the BOREXINO Project. Essential features evolve
since the original Brookhaven lines and the GALLEX/GNO system used in germane synthesis.
The engineering picture portrays the appearance of the counting system for the gas proportional counter
set-up. The system is encased in a Radon Box cover (not shown) which is used to provide a controlled atmosphere environment
along with an air lock for the insertion of counters. Shown here is one gas counter copper box inserted into a counting hole.
The counter box is in a copper block made from specially shipped NOSV copper from Nord Deutsche Affinerie, currently stored
underground at MPIK. The lead housing is selected from low background lead. The NRL group's engineers and scientists handle
this piece of the project with MPIK engineering design assistance.
Achievements of the project
The project was officially ended in 2006. The following has been achieved:
- Construction of the ultra-low background Ge spectrometer (GeMPI II) operated now at the Gran Sasso laboratory
- Construction of 6 low-background miniaturized proportional counters
- Construction of the counting system for the gas proportional counters
- Design study of a new counter filling line
References
[1] Underground Ultra-Low Background Micro-Chemistry and Counting at Gran Sasso: The M-Cavern Project, LNGS-LOI 31/03
[2] H. Neder, G. Heusser, M. Laubenstein, "Low level gamma-ray germanium-spectrometer to measure very low primordial radionuclide
concentrations", Appl. Radiation and Isotopes 53 (2000) 191-195.
[3] C. Schlosser, "Germanium-Ausbeutekkontrolle und Charakterisierung von Untergrund beit ragen in Gallex Sonnenneutrino Experiment",
Universitaet Heidelberg and MPIK, Heidelberg (1992).
[4] R. Wink et. al. incl. G. Heusser, T. Kirsten, Nucl. Inst. and Methods in Phys. Res. Ser. A 329 (1993) 541.
[5] W. Hampel et. al., (GALLEX Collaboration), "Gallex Solar Neutrino Observations: Results for Gallex III)", Phys. Lett.
B388 (1996) 384-396.
[6] H. Simgen, Diploma Thesis, MPIK and University Heidelberg (2000).
[7] S. Pezzoni, "Non solar-neutrino production of 71Ge in Gallex", PH.D. Thesis, Max Planck Instutut fur Kernphysik,
Heidelberg MPIH-V2-1995 (1995).
[8] H. Simgen, Ph. D. Thesis, MPIK and University Heidelberg (2003).
People involved: